Keywords
Abstract
The review gives a systematic analysis of published data devoted to multisensor technologies for manufacturing effective miniature devices capable of simultaneous accurate determination of several analytes and/or characteristics of biological fluids. The use of electrolyte-gated field-effect transistors as a biosensing platform is considered in detail. The devices based on these transistors demonstrate record-low limits of detection and are non-complicated for mass production. The use of electrolyte-gated organic field-effect transistors in combination with aptamers capable of specific binding to various analytes paves the way to innovations in early medical diagnostics (label-free biomarker detection). The most successful examples of multisensor devices using these transistors are presented, and the prospects of using aptamers as recognition elements in these devices are demonstrated.
Bibliography — 154 references.
1. Introduction
In recent years, efforts of numerous scientists and engineers have been directed towards development of approaches to multi-target biological analysis, which implies parallel determination of one or (simultaneously) several analytes using electronic and optical methods. Devices able to recognize several parameters in different media are called multisensors.
The idea of designing multisensor systems is not new; it was proposed for the first time in the second half of the 20th century for gas sensors capable of multiple detection in a single analysis[1]. The propagation of this idea resulted in the design of devices currently known as electronic nose (E-nose)[2]. Multisensors pave the way to the possibility of measuring and analyzing a large amount of data with simultaneous determination of various parameters of the medium. These devices, able to process arrays of responses in a short time, provide more accurate results. A large number of reviews on various multiplex devices have been published. Some of them describe particular sensor architectures[3, 4], while other ones focus on the general principles of operation of multisensor systems and description of these principles[5, 6].
Currently, studies concerned with biocompatible multisensors are actively developing, including new detection methods and approaches to sensor design. The major effort is concentrated on the design of effective miniature devices capable of accurate simultaneous determination of several analytes in liquid media, including biological fluids. Many reviews are devoted to classification of biosensors based on various characteristics such as combination of bioreceptors, diversity of transducers, materials, detection systems and so on[7-11].
An electrolyte-gated organic field-effect transistor (EGOFET) is a modern device promising for the use in biosensing: sensors based on these transistors are characterized by low operating voltage and very high sensitivity[8, 12, 13]. The EGOFET-based technology combines a number of benefits such as selectivity, fast result and small size of the devices; the last-mentioned issue allows for the manufacturing of portable biosensor systems to determine either one or a few analytes simultaneously[14-17]. Publications dealing with EGOFET-based biosensors often appear in the foreign scientific journals. A number of reviews[10, 18, 19] are devoted to possible applications of EGOFETs as biosensors, approaches to their fabrication and types of recognition elements used. However, no general publications on EGOFET-based multisensors are available to date, and the present review is intended to fill this gap.
A variety of approaches are used for EGOFET biofunctionalization, e.g., modification of the gate or the organic semiconducting layer using various recognition elements of the sensor. One of the options for recognizing elements are aptamers, which proved to be effective in the development of a broad range of sensing devices, owing to their adaptability to determination of various targets, remarkably low limits of detection (LoD) and broad analytical range of quantitative determination[20]. The use of aptasensors for multiplex analysis has been surveyed in a number of publications[21-26]. Today, in view of these benefits, studies dealing with the design of biosensors based on EGOFET architecture using aptamers as the recognition layer are actively carried out.
The present review addresses the modern EGOFET-based biosensors and discusses the potential of using these biosensors as a platform for the design of multisensor devices with aptamers as recognition elements.
2. Classification of biosensors
The classification of biosensors may vary depending on their basis. Thus, sensors can be split into groups in terms of the nature of recognition elements, procedures for measuring the response or the type of device used[27]. In this review, we give classification of biosensors used to design devices for point-of-care diagnostics (РОС devices).
In the general case, modern biosensors can reasonably be classified in terms of the following characteristics (Figure 1):
— the use or no need to use labels for analysis;
— the way of recognition (enzymatic, non-catalytic protein and immune sensors; DNA biosensors and whole-cell biosensors);
— type of the output signal (electrochemical, optical, thermal and mass-sensitive sensors);
— multiplexity (multisensors, selective sensor arrays, electronic noses as arrays of semi-selective sensors, labs-on-chip).
The recognition elements present in biosensors can be subdivided, in terms of operation mechanism, into affinity and catalytic types[28, 29]. Catalytic devices contain enzymes, cells, or tissue slices that recognize the target analyte and produce electroactive substances. In the affinity type biosensors, only affinity type non-covalent interactions between the recognition element and the biomolecular target take place; this induces changes in a physical or physicochemical characteristic of the recognition layer that can be measured. Antibodies, receptor ligands, nucleic acids and other compounds, including aptamers, are used as recognition elements. The possible target analytes include low-molecular-mass compounds (including ions), proteins, viruses or microorganisms as well as drugs, hormones, toxins, etc. The broad range of target analytes provides the great variety of biosensor applications ranging from medical diagnostics and veterinary medicine to food industry, agriculture and biosafety.
2.1. Classification by use of labels
In terms of the analyte detection principle, biosensors are generally subdivided into two large types:
— label-based biosensors, i.e., those using labels preliminarily introduced into the analyte;
— label-free biosensors.
A label is any foreign molecule that can be detected at a much lower concentration than the analyte. The range of possible labels includes chromophores (spectrophotometric determination), fluorophores (measurement of the fluorescence spectrum or fluorescence intensity at characteristic wavelengths), electrochemical species (determination by electrochemical sensors), radioactive species (determination of β- or γ-particles using scintillators or photosensitive materials) and so on[30, 31]. The label-based methods are fairly accurate and provide low limits of detection and a broad analytical range of determination; however, they also suffer from significant drawbacks: label-based techniques are more complicated than label-free ones, they are labour-consuming, first of all, this refers to the process of labelling. Moreover, they require elimination of side reactions that accompany the introduction of labels and elimination of the effect of labels on analyte binding.
The operation principle of label-free biosensors is the use of physicochemical interactions and properties of an analyte for detection. This method is most promising for the design of biosensors if a highly sensitive analytical method is used. The advantages of label-free methods include:
— high performance,
— ease of analysis,
— simplicity of integration on a substrate,
— low cost of the devices manufacture.
An example of highly sensitive analytical method for label-free biosensors is the procedure called ‘single molecule with a large transistor’ (SiMoT) developed in 2018[32]. For electrolyte-gated biosensors, this approach provided a decrease in the limit of detection down to zeptomolar concentration level. In essence, this means that a single molecule can be detected in 1 mL of a solution. However, the complexity of sample preparation and relatively narrow concentration range (up to a few nanomoles per litre) restricts the applicability of this approach outside a laboratory.
2.2. Classification by the recognition element
2.2.1. Enzymatic biosensors
The operation of enzymatic biosensors is based on direct or reverse enzyme-catalyzed transformations of a neutral substrate into an analyte that can be quantified either electrochemically or optically. Many enzyme-catalyzed reactions involve the release or uptake of an analyte, and its amount can be measured with a suitable sensor coupled to the immobilized enzyme. Enzymes can also be conjugated with recognition elements, and this expands the range of potential analytes. The choice of the enzymes in the biosensors is conditioned by the analyte to be detected. The biosensors make use of reactions involving enzymes such as oxidoreductases, isomerases, lyases, ligases, transferases and hydrolases[33]. An alternative method is to determine the analyte using mediators as oxidants and electron transfer agents, which allows efficient operation even at low potentials irrespective of the oxygen content. The mediators used in biosensor systems include ferricyanide, ferrocene, methylene blue, phenazines, methyl violet, alizarin yellow, Prussian blue, thionine, azures A and C, toluidine blue or inorganic ions (oxidizing and reducing agents).
2.2.2. Non-catalytic protein biosensors and immunosensors
The operation of biosensors based on protein receptors, or non-catalytic protein biosensors, is based on the ability of proteins to perform recognition. This type of sensors may use both receptor proteins that mimic the biosensing properties of cells and other types of proteins able to form complexes with the analyte. A popular option is to use antibodies as recognition elements. The design of most immunosensors is based on the solid-phase immunoassay principle in which the antigens or antibodies are immobilized on a solid substrate[30, 34, 35]. The antigen — antibody interaction takes place at the solid/liquid interface. The main advantages of immunosensors are their high sensitivity and specificity.
2.2.3. Aptamer-based biosensors
Biosensors based on DNA aptamers have been developed as an alternative to antibody-based biosensors; they are characterized by high structure stability, specificity and low prime cost[36-38]. The biosensors based on DNA aptamers can selectively bind to bacteria, viruses, proteins, hormones and even to small molecules and ions with high specificity and high affinity. This recognition mainly involves hydrogen bonds, electrostatic interactions and hydrophobic effect. The development of new aptamers resulted in the design of novel biosensor devices characterized by high stability and specificity, lower cost and much simpler detection principles compared to those in immunosensors.
2.2.4. Whole-cell biosensors
Biosensors based on reporter genes are widely used in medicine. A genetically engineered whole-cell biosensor utilizes prokaryotic or eukaryotic cells to determine the chemical composition, toxicity, carcinogenicity and mutagenicity. Whole-cell biosensors may be designed by genetic engineering. For example, the gusA gene encoding β-glucuronidase was used as an inserted marker gene to manufacture several enzymatic biosensors[34].
A widely used reporter gene is gfp, which encodes the green fluorescent protein (GFP). The gene expression is used to visualize various bacterial strains by confocal microscopy, wide-field fluorescence microscopy and flow cytometry[39]. A change in the fluorescence in these biosensors is associated with expression inhibition, cell death or, in more complex systems, with analyte-dependent change in the GFP expression[40].
2.3. Classification by the signal processing method
Depending on the method of signal transmission and processing, biosensors can be subdivided into optical, mass-sensitive and electrochemical ones.
2.3.1. Optical biosensors
Optical biosensors are the most often used type of biosensors. The response in these sensors appears when optical radiation interacts with the biorecognition element. Like electrochemical biosensors, optical biosensors are subdivided into label-based and label-free ones[41].
The action of optical biosensors may be based on several optical signal transduction methods, including fluorescence, phosphorescence, surface plasmon resonance (SPR), etc. Back in 2007, SPR-based optical biosensors demonstrated the possibility of label-free determination of a single molecule in a solution[42]. The drawbacks of this method include high cost of the devices and the difficulty of performing analysis outside a laboratory.
2.3.2. Mass-sensitive biosensors
Mass-sensitive biosensors detect an analyte via monitoring and recording the changes in the resonance frequency (e.g., microbalance biosensors in which a quartz resonator acts as the sensing element), deviation of a beam (e.g., deviation of a laser beam from the cantilever tip in optical arm microcantilever sensors) or electrical resistance of the sensor caused by the mass of analyte molecules bound to the sensor surface.
The main benefits of mass-sensitive biosensors are real-time operation and the possibility of monitoring in liquids, air and vacuum. Nevertheless, most of these biosensors are rarely used, because they employ piezoelectric materials as signal transducers, and, hence, the sensitivity of such devices is usually lower than that of SPR-based devices. One type of mass-sensitive sensors are microcantilever biosensors, the attractive features of which are lower limits of detection compared to those of classical methods and a very small size (<10–3 mm2); this means that very small amounts of both the receptors and the analyte are required for the assay[43].
2.3.3. Electrochemical biosensors
The operation of electrochemical sensors is based on the interaction with the target analyte and generation of an electrical signal proportional to the analyte concentration.
Electrochemical biosensors are cost-effective, portable, highly sensitive and compatible with modern microfluidic technologies[44]. They can be used to fabricate inexpensive and convenient devices for detection of various analytes and are widely applied in a number of fields. The popularity of the electrochemical method of detection is due to the following main benefits:
— a large selection of signals that can be measured such as voltage, current, total output power or electrochemical impedance,
— low theoretical limits of detection, which are dictated by differences between the Faradaic and non-Faradaic currents.
2.4. Classification of multisensor devices
Biosensors possess a great potential for multiplexity. This means that these devices can detect a few analytes simultaneously; this has a beneficial effect on the cost, quality and time of analysis[45, 46].
Currently, there is no well-established classification of multisensory devices: any device comprising more than one sensor can be called multisensor. However, in the general case, multisensors can differ in the selectivity of the used sensors to the target analyte and integration scale of the manufactured devices. In addition, there are established names for liquid and gas sensor arrays: electronic tongue (E-tongue) as an analogue of the human tongue, and electronic nose (E-nose) as an analogue of the human nose.
A variety of approaches to the design of multisensory devices have been developed. In terms of this criterion, they can be divided into several classes:
— an array of sensors to detect only one characteristic,
— e-tongue as an array of sensors with low selectivity supplemented by machine learning or artificial intelligence to determine simultaneously several characteristics,
— lab-on-chip, in which the primary signal processing circuit is integrated on a sensor substrate and recognizes several analytes at once.
In terms of selectivity, multisensors can be divided into arrays of fully selective sensors in which each sensor or a group of identical sensors is responsible for measuring a particular parameter[47] and arrays consisting of semi-selective sensors (e-tongue) in which measurements are implied to generate so-called fingerprint of the analyte, instead of a particular response to the analyte. Machine learning processing of this fingerprint makes it possible to unambiguously determine the presence of the target analyte.
An array of several sensors to be immersed into an analyte solution can be fabricated on various substrates; this allows detection of different input response parameters and processing of output signals by means of a single controller. As a result, one or several output characteristics can be determined with high accuracy. The benefits of such devices are easy manufacture and high reliability of the results, because the error of one sensor is minimized due to the presence of other sensors and also because of partial independence of the sensors[47]. The e-tongue is an analytical device consisting of a set of chemical and/or biological sensors with low selectivity, possessing cross-sensitivity, and capable of simultaneous detection of several components in the analyte solution. The data are processed using a variety of multidimensional approaches such as image recognition, calibration, machine learning or neural networks.
The integration scale conventionally means the replaceability of particular parts of the device. Thus, the controller of a multisensor can be designed as a separate element, or it can be integrated on the substrate or arranged as a separate sensing layer. The sensing elements of the multisensor can be mounted on separate substrates and can be interchangeable, or they can be manufactured by printing on a single substrate, which markedly facilitates the manufacturing process. The modern trends dictate the simplification of the manufacture, which has resulted in the appearance of so-called lab-on-chip devices.
A lab-on-chip is any sensor system mounted on a single substrate with an integrated microchip, which serves for processing and transduction of signals from the sensors[48]. These devices are fabricated on a single small substrate with the area ranging from a few square millimetres to a few square centimetres (Figure 2). The advantages of these sensors are the miniature size, which makes it possible to use them in POC devices and to use very small volumes of the analyte[49], and potentially low cost of manufacturing with simple scalability[50], because the whole device can be fabricated on one substrate by various lithography methods.
The fabrication of multisensors would be impossible without the development of printing technologies to make inexpensive, non-invasive, environmentally benign, disposable and wearable diagnostic tools of new generation for continuous monitoring of analytes in biological fluids such as, for example, saliva.
Bihar et al.[51] described a sensor completely printed on paper (Figure 3); this device has a high sensitivity to glucose present in saliva in concentrations typical of diabetes mellitus patients. The shelf life of this sensor is at least a month. The authors used glucose as a target analyte; however, the range of substances detectable with this platform can be expanded quite easily just by changing the recognition enzyme, and thus a printed multisensor can be fabricated. The described device consists of two printed layers of the poly(3,4-ethylenedioxythiophene) complex with polystyrene sulfonate (PEDOT : PSS), which are used as the reference, working and counter electrodes. The proposed technological platform can include a variety of recognition elements, which provide both the multifunctional character of the device and a wide range of its applications. A key benefit of this product is that after integration with wearable biocompatible substrates, this device can be adapted to provide continuous monitoring of glucose level in saliva.
The benefits of multisensors include the possibility of simultaneous detection of several parameters in one sample over a short period of time with high accuracy and miniature size of the whole device. The appearance of multisensors can be regarded as an evolutionary step related to the engineering development of monosensors.
Thus, there are quite a few classifications of biosensors based on the nature of the recognition elements, method of measuring the response, method of analyte detection, etc. One of the approaches used for analyte detection is the insertion of a fluorescent or electroactive label. However, this approach is resource-consuming; therefore, label-free detection methods are used more and more often.
3. Electrolyte-gated organic field-effect transistors: architecture of devices, advantages and approaches to fabrication
Electrolyte-gated organic transistors (EGOTs) are electrical sensing devices suitable for the label-free detection of various analytes, EGOTs are the products of ion-selective field-effect transistors (ISFETs) evolution. In these devices, the ion selective membrane (the key element of ISFET) is replaced by a layer of semiconducting material coated by a recognition layer, which allows for the use of signal amplification methods typical of field-effect devices and thus extends the range of applicable concentrations (molecular masses) of analytes.
There are two types of EGOTs: electrolyte-gated organic field-effect transistors (EGOFETs) and organic electrochemical transistors (OECT). The EGOFET devices are based on organic semiconducting films which are impermeable for ions (Figure 4). Applying a voltage between the gate and source (VGS) leads to accumulation of ions at the interface between the electrolyte and the gate and at the interface between the semiconductor and the electrolyte. This results in the formation of a high-capacitance electric double layer (EDL). In this case, the interfaces of EGOFET act as capacitor plates. The measured capacitance of these capacitors is much higher for EGOFETs than for other types of sensors and may reach tens of microfarads per square metre.
If in addition to the voltage applied between the gate and the source, there is a voltage applied between the source and drain electrodes (VDS), the field-induced charge carriers accumulated in the organic semiconductor will induce current flow (IDS) in a similar way as in inorganic field-effect transistors (see Figure 4). The materials used in EGOFET devices include pentacene, P3HT, pBTTT-C14, etc[53, 54].
Organic electrochemical transistors operate by a somewhat different mechanism: in these devices, current arises due to the change in the charge of the semiconductor itself, which is reversibly oxidized under the voltage applied to the gate (VG). Currently, typical semiconducting materials for the use in OECTs are PEDOT : PSS, p3CPT, p(h2T-TT), etc[55, 56].
Thus, a distinctive feature of EGOFET devices is the presence of a layer of organic semiconductor, which is not doped and acts as a functional (conductive and recognition) layer. To achieve high selectivity, the surface of a sensor can be modified with various biorecognition layers; modification can be applied to the surface of both the semiconductor and the gate. Various designs of EGOFET devices are shown in Figure 5.
In general, the following benefits of EGOFET devices can be listed:
— low limits of detection: EGOFET-based sensors are capable of responding to an analyte present in down to zeptomolar concentrations[32];
— low operating voltage: EGOFET-based sensors operate at voltages significantly lower than those in the organic field-effect transistors (0 to –0.5 V); this is a key feature, as this makes it possible to avoid redox and other undesirable reactions in the aqueous solution of the analyte and on the electrode surface[13];
— the possibility of the device fabrication on flexible substrates: the choice of the substrate material is not a crucial factor for EGOFETs as long as it is made of a dielectric; this opens up the possibility of using EGOFET sensors in flexible electronics and significantly expands the scope of their applications and methods of fabrication[57];
— there is no need to introduce special labels to detect the analyte; this markedly facilitates the analysis and obtaining the result and eliminates undesirable reactions in the solution as well.
According to Kergoat[58], EGOFET device is a sensor in which no doping — dedoping of an active layer takes place and which shows a response owing to the amperometric changes that arise upon the change in EDL under action of an analyte (as opposed to OECT). In fact, the differences between OECTs and EGOFETs are rather conventional, and the mechanisms of their operation even in theoretical studies are based on the same Shockley, Helmholtz and other approximations. When layers of a new semiconducting material are used, it is often impossible to find out exactly whether or not doping takes place in the material, or the semiconducting layer coated by the dielectric layer is still affected by electrolyte ions. In order to avoid mistakes in the terminology, various research groups use different descriptions while designing new unusual devices. For example, Massey and Prakash proposed the acronym OEGFET (organic electrolyte-gated field-effect transistor), instead of OECT, i.e., they implied that the devices operated according to the principles characteristic of FETs, although a typical semiconducting material in these devices was PEDOT : PSS. The architectures of EGOFET and OEGFET are depicted in Figure 6. Other researchers do not use such classification and consider the devices they design just as electrolyte-gated organic transistors[12, 59-61].
The EGOFET operation depends, first of all, on the composition and morphology of its active layer. In the general case, this layer does not undergo doping — dedoping under the action of the applied potentials and the current through the system cross-section is directly correlated with the surface thickness and roughness. A smooth layer rules out the appearance of charge traps at the interface, while the layer thickness directly influences the charge carrier injection from the semiconductor surface to the electrode. Currently, there are several approaches to fabrication of the active layer for EGOFETs. Solution methods such as inkjet or patterned printing, the doctor blade technique, etc., can be used to deposit relatively thick layers of organic semiconductors from solutions; however, these layers may be not sufficiently smooth or may have non-uniform thickness[10]. Low-temperature vacuum thermal evaporation is a better method, but it is more complicated in implementation; according to this method, the vapour generated by high-vacuum evaporation of the desired material is deposited on the electrode, with the thickness of the resulting layer being controlled. Drawbacks of this approach include the engineering complexity (equipment for high vacuum generation is necessary) and the relatively narrow range of molecular masses of compounds for deposition (low-molecular-weight compounds are not always deposited, while high-molecular-weight compounds often decompose at temperatures below the sublimation temperature).
One more promising trend is the fabrication of self-assembled semiconducting and/or recognition monolayers, which can be obtained, for example, using the Langmuir – Schaefer or Langmuir – Blodgett techniques or by self-assembly from solutions (Figure 7).
Thus, EGOFETs are a subclass of EGOTs. EGOFETs differ from similar OECT sensors by the charge transfer mechanism in the semiconductor channel; however, the boundary between these architectures becomes more and more uncertain. There are various methods for manufacturing EGOTs; the use of monolayers for the formation of semiconducting or recognition biolayer is considered to be promising. The possibility of fabrication of ultrathin semiconducting layers and the use of amplification methods typical of OFET devices made it possible to implement EGOFET-based technology for detection of a single molecule (SiMoT).
4. Detection of a single molecule with a large transistor
The conceptual possibility of detecting a single molecule in solution was demonstrated back in 2007.42 This possibility was implemented using optical methods and microfluidic toroidal resonators. Binding of molecules resulted in a change in the resonant wavelength near the toroid.
A promising technology related to the EGOFET architecture is SiMoT, that is, detection of a single molecule with a large transistor (Figure 8), which was first described by Macchia et al.32 This method, like (most often) EGOFET devices, is based on semiconducting self-assembled monolayers (SAMs) and a biorecognition layer. The analyte binding in the active layer of these devices occurs very quickly (within a few minutes) even at very low concentrations. This platform demonstrates very low limits of detection in label-free assays and possesses a tremendous potential for label-free detection of proteins and biomarkers in early medical diagnostics.
The device responds to the threshold voltage shift induced by a change in the analyte concentration (Figure 9а). Figure 9b shows the correlation between the SiMoT threshold voltage shift to more negative potentials and a decrease in the gate work function in each functionalization stage and after affinity binding. The decrease in the work function is caused by the electrostatic effect of the dipole that is oriented along the z axis, while being attached to the x – y gate surface and pointing away from the surface with the positive pole.32
Later, Di Franco et al.[63] described the mechanism of SiMoT technology considering measurements of the surface potential of the biofunctionalized electrode. The measurements showed that binding of only ten (or less) Ig molecules induced a change in the surface potential in 108 capture anti-IgM antibodies present as the recognition layer. The authors proposed the following amplification mechanism. The propagation of electrostatic (dipole) changes caused by affinity binding to one capture antibody eventually affects numerous other antibodies according to the domino effect. Actually, even a weakly physically adsorbed layer is effective. This means that electrostatic interactions between well-packed capture antibodies segregated on the gate surface can promote propagation of electrostatic changes that occur on a single capture antibody.
Owing to the demonstrated capability of detecting single molecules using monolayers in biosensor devices, studies of these systems has received increasing attention[64]. Tricase et al.[65] used cyclic voltammetry and X-ray photoelectron spectroscopy to study the conformational rearrangement of a self-assembled N-(2-hydroxyethyl)-3-mercaptopropanamide monolayer on the surface of the gate electrode after application of an electric field depending on the protocol of SAM synthesis. Three protocols were developed, one of which provided the most efficient strategy for electrode modification affording a surface coating by SAM with a density of 1.29 × 1013 molecules per cm2. It was found that application of an electric field during the measurement of the EGOFET sensor response induces a conformational rearrangement of SAM due to strong hydrogen cross-chain bonds formed by NH and COOH groups. Probably, this rearrangement is responsible for the domino effect, which accounts for the ultrahigh sensitivity of SiMoT[65].
Currently, the technique of a single molecule detection, which proved to be efficient, becomes more and more popular in the biorecognition[66-70]. For example, Sun et al.[71] were able to reproduce the SiMoT protocol and attained a recognition limit of 4.75 × 10–18 М. The authors used an OFET-based multisensor system with pillar[n]arene for signal amplification. They performed simultaneous determination of α-fetoprotein, cancer embryonic antigen and prostate-specific antigen, which is considered to be a key issue for early cancer diagnosis[71].
Thus, using SiMoT technology, it is possible to detect extremely low concentrations of various bioanalytes by field effect-based sensor devices. This technology is perfectly combined with the EGOFET architecture, the main advantages of which are low limits of detection and operation at low gate voltages.
5. Diversity of modern biosensors based on electrolyte-gated organic field-effect transistors
As was noted above, the EGOFET-based sensors are used more and more often for biorecognition; therefore, the range of these devices and their applications is also expanding. A number of reviews[10, 18, 19] are devoted to the use of EGOFETs as biosensors. In general, EGOFET sensors can be classified according to the following criteria:
— the type of analyte (viruses, DNA elements, proteins, hormones, cells, etc.);
— approach to active layer functionalization (organic semiconductors, aptamers, antibodies, small molecules, polymers, etc.);
— method of response measurement (amperometry, potentiometry, coulometry, etc.).
EGOFET devices are suitable for determining not only bioanalytes over the whole range of their molecular masses, but also physicochemical parameters of biological fluids such as pH (Figure 10)[72]. It is noteworthy that in this case no redox reactions take place on the surface of the device (due to the low gate–source voltage, VGS = –0.5 V).
Using EGOFETs, it is possible to successfully determine analytes with a relatively low molecular mass, in particular vitally important compounds such as dopamine (153.2 Da)[73], cortisol (326.5 Da)[59] and other hormones; owing to different mechanisms of analyte binding, EGOFETs can determine not only ions but also neutral molecules[74].
The development of new approaches to functionalization of the biorecognition layer brought about the possibility of determining large molecules, in particular procalcitonin (14.5 kDa)[35] and α-synuclein (14.46 kDa)[34]. Gate functionalization with antibodies and aptamers (Figure 11) allowed for the detection of DNA and RNA elements and also viruses with high sensitivity[36, 75-77]. For example, Selvaraj et al.[76] demonstrated the capability of EGOFET sensors for the label-free determination of short sequences of genetic material in relation to the detection of micro-RNA-21. The EGOFET surface was modified by the thiol derivative of a complementary oligonucleotide that specifically binds to micro-RNA-21. The presence of response, that is, a change in the voltammetric characteristics of the device, was demonstrated; surface binding was confirmed by impedance spectroscopy and SPR. Theoretical substantiation of the response as a result of surface modification was also presented.
Using EGOFET it is possible to evaluate the results of immunotherapy. The efficacy of this therapy can decrease due to the development of immune response against the drug, which may result in the generation of anti-drug antibodies (ADA) in treated patients. Sensi et al.[75] reported the first EGOFET-based immunosensor for the label-free detection of ADA. As a compound (molecule) for specific recognition, they integrated the biological drug nivolumab for quantitative and selective detection of ADA against this drug. The nivolumab attachment to the gold surface was performed with an anchor represented by Cys-protein G, while non-specific interactions were minimized using 11-mercaptoundecyl triethylene glycol (see Figure 11). The limit of detection by this immunosensor was 100 fM. The developed approach demonstrates the possibility of detecting ADA under clinical conditions for timely correction of therapy in the case of secondary drug insensitivity and can also serve for fast screening of biological drugs for immunogenicity.
Determination of molecular ions deserves special attention. Benasco et al.[78] demonstrated the possibility of detecting phosphate ions by EGOFET-based sensors. The device channel contains a composite layer consisting of a diketopyrrolopyrrole-based semiconducting polymer and cyanostar, a π-conjugated penta-tert-butylpentacyanopentabenzo[25]annulene receptor, able to recognize oxyanions. This design demonstrates a novel concept in which the receptor synergistically enhances the stability and transport characteristics through doping.
Wustoni et al.[79] implemented a similar idea, but using crown-based compounds, with the OECT architecture for the detection of sodium and potassium ions (Figure 12). The polymer films containing thiophene units functionalized with a crown ether specific to either Na+ or K+ generate an electrical response proportional to the type and concentration of the cation. Ion-selective polymers are used as the OECT gate electrodes the current of which varies depending on the concentration of ions to which the electrode is selective. An organic electrochemical transistor designed as a single miniature chip provides selective detection of cations in the physiologically significant range of concentrations. These electrochemical ion sensors require neither ion-selective membranes nor reference electrodes and may potentially surpass the existing techniques for detecting alkali metal ions in aqueous media.
The detection of markers of various diseases, in particular HIV infection[80] and dangerous neurological disorders that precede such pathologies as dementia and Parkinson’s and Alzheimer’s diseases[81-83], has now become possible using EGOFET-based sensors. It is noteworthy that even without using SiMoT technology, the designed biosensors have rather low (10–15 M) limits of detection and high reproducibility of the results. When the SiMoT technology is used, the detection limit of EGOFET devices can be lowered to zeptomolar values. This means that such a device can detect a single analyte molecule in 100 mL of a solution[84].
Poimanova et al.[85] described a portable flow type EGOFET sensor with the biorecognition layer based on aptamers (Figure 13). The fabricated devices demonstrated a highly selective response to influenza A virus over a wide range of concentrations (6 × 104 – 6 × 108 viral particles in 1 mL) comparable with the concentration range in real biological specimens. In addition, for increasing the accuracy of analysis, the possibility of simultaneous signal acquisition from several sensors is envisaged in the fabricated prototype.
The functionalization of the sensor active elementѕ plays a crucial role in the device operation. There are several approaches to fabrication of the active layer. The semiconductor and biorecognition layers can be
— deposited from a solution using the doctor blade method[86], spin-coating[87] or inkjet printing[88, 89];
— transferred from the surface of a liquid (water or another solvent) by the Langmuir – Schaefer or Langmuir – Blodgett methods.
These methods make it possible to fabricate semiconducting films with a thickness of approximately several nanometres and form thin SAMs on the EGOFET surface. The structure and morphology of the SAM are the key parameters for EGOFET, which primarily determine its voltammetric characteristics.
Not only solutions, but also gels can be used as electrolytes for EGOFET operation. For example, Pallu et al.[90] described DNA-based bulk hydrogels, which were grown on the EGOFET gold gate electrode. It was shown that the modified device retains the electrical characteristics typical of electrolyte-gated devices and that the transistor can operate without the addition of external electrolyte, namely as a solid-state hydrogel-gated device. This is the first example of using a bulk functional DNA hydrogel as a solid-state electrolyte in EGOFETs.
Studies dealing with theoretical substantiation of the of operation of EGOFET-based sensors are in progress. Huetter et al.[91] considered an analytical and physical model for determining the voltammetric characteristics of EGOFETs in the Helmholtz approximation using only the physical parameters of the device. On the basis of this model, analytical expressions for phenomenological quantities characterizing the ideal model of a field-effect transistor were derived in terms of the physical parameters of the devices. Delavari[92] performed computer simulation of EGOFETs. This was the first case where EGOFET model was studied using the Nerst – Planck – Poisson equations for the description of both polymeric and electrolytic parts of the device architecture.
In recent years, more and more multisensor EGOFET devices have appeared. Doumbia et al.[93] developed a label-free biosensor consisting of a set of EGOFETs integrated with a multichannel microfluidic platform on a flexible substrate. The device showed reproducible electrical characteristics and could operate at various electrical voltages for more than an hour, while retaining 85 – 96% of the initial performance. The applicability of the biosensor for real-time monitoring was demonstrated by in situ and operando detection of DNA hybridization with good reproducibility and selectivity and with a temporal resolution of less than a second in a flowing electrolyte. The developed platform, unlike previous EGOFET-based biosensor platforms, did not require the incubation step or purification to remove non-specifically adsorbed molecules. The EGOFET-based sensor technology is increasingly commercialized. The fully flexible OEGFET described by Massey and Prakash[94] is the first example of a electrolyte-controlled OFET biosensor with integrated soft microfluidic channels that was tested using both synthetic saliva samples and saliva samples containing impurities. As the benefits of the described technology, the authors mentioned easy manufacturing of the devices: they used an inexpensive low-temperature method of functional layer deposition and a flexible substrate, which makes the resulting devices versatile[94-102].
To summarize the foregoing, we present a comparison of various sorts of EGOT biosensors in terms of the limits of detection, operation media, and target analytes (Table 1).
Thus, quite a number of types of EGOT-based biosensors operating in a wide variety of media for determination of numerous analytes have been described to date. However, it should be noted that determination of a single molecule in solution is quite a challenging task, since, as a rule, the response to a single molecule is in the range close to the limit of detection, and reliable measurement is not always possible due to the presence of noise.
6. Multisensors based on electrolyte-gated organic field-effect transistors
Modern multisensor technologies are being actively developed, with single sensors being incorporated in full-scale systems capable of highly accurate recognition of various analytes or a single analyte. Biosensors make no exception to this trend. The progress in the field of printing technologies and appearance of wearable electronic devices greatly simplified the fabrication of multisensor systems. Indeed, glucose sensors printed on paper showed high efficiency and low limits of detection (approximately a few micromoles in 1 litre) (Figure 14)[96].
Currently, multisensor devices are successfully manufactured on OECT (Ref. [103]) and OFET (Ref. [104]) platforms. This refers to all types of sensors that are suitable for commercial applications, including EGOFET-based sensors. A distinctive feature of EGOFET-based sensors is high sensitivity to target analytes, which makes them especially promising for early medical diagnostics. These devices are capable of highly accurate and fast detection of characteristic markers of diseases and disorders that often cannot be detected by conventional analysis[52, 68].
Multisensor devices are divided into various classes depending on the type of sensor and method of sensor manufacturing (Figure 15). This review addresses methods for manufacturing of multisensor devices that are applicable to EGOFET-based sensors.
EGOFET-based sensors are manufactured using relatively thin films of organic semiconducting materials sensitive to external stimuli and self-assembled recognition biolayers on the surface. The thin recognition biolayers do not damage the semiconductor surface and, apart from functionalization, they perform the laminating function to eliminate the influence of external electrolyte. In addition, this approach makes it possible to deposit several biorecognition layers. A few logical approaches to the fabrication of EGOFET-based multisensor devices have been proposed. Some of them are listed below.
1. Sensitive elements of a multisensor can be mounted on one substrate and then split into cells to generate an array of sensors[105]. The main advantage of this approach is the ease of fabrication of sensors, which can together give a more accurate assay results owing to higher signal-to-noise ratio for the whole device.
2. Sensitive elements of a multisensor can be mounted on one substrate and then split into cells and modified with various biorecognition layers to obtain a multisensor for simultaneous recognition of several characteristics.
3. Lab-on-chip is a type of device in which various computing circuits can be printed directly on a substrate with a recognition layer. Using this approach, it is possible to reduce the sensor size (achievements in microfluidics are actively used in this design) and also to simplify the manufacturing process: integrated circuits are easy to manufacture and represent miniature chips (Figure 16)[106].
One more approach to the design of EGOFET-based multisensors is the formation of several modulated gates. Scandurra et al.[107] added a reference electrode to the device, apart from the sensing electrode; this enabled potentiometric measurements. As compared with single-gate EGOFETs, the manufactured device has more functions; however, in operation of the device, the possible influence of parasitic Faradaic currents should be taken into account.
The subsequent development and improvement of these devices largely depend on the choice of electronic circuits for signal transduction and processing, which should most fully exploit the potential of the sensors by means of circuit designs adapted to sensor characteristics.
The solution of this problem is no less important than formation of the multisensor active layer; however, it is rather related to electronics, therefore, it will be briefly considered by the example of only one study[14], in which the authors designed a transimpedance amplifier to convert the current of the EGOFET-based sensor into an output voltage (Figure 17а). The need to develop a nontrivial amplifier circuit is due to the fact that in the case of conventional current-to-voltage circuits (Figure 17b,c), an acceptable circuit stability and time and resolution characteristics (signal bandwidth and gain) cannot be achieved because of the intrinsic parasitic capacitance of the sensor. The authors were able to solve these problems by developing an adapted analog electronic circuit and to obtain a front-end signal converter with characteristics independent of the parasitic capacitance of the sensor. This allowed an adequate readout of the signal and significantly simplified further signal processing.
In addition, Genco et al.[14] proposed a multisensor device for early detection of pancreatic cancer. The authors described a multiplexed current-sensitive readout device for the label-free zeptomolar-sensitive detectors immobilized on large-area EGOFETs. These highly capacitive biosensors are multiplexed using organic thin-film transistor (OTFT) line driver and OTFT switches and interfaced to a low-power silicon front-end integrated circuit manufactured by CMOS 65-nm technology (CMOS is complementary metal oxide semiconductor) sensitive to picoampere currents. The integrated circuit also performs analog-to-digital conversion and data transmission to a microcontroller. An OTFT-based current conversion circuit with a power supply of 30 V is used for the transmission of signals arriving from several biosensors to the CMOS circuit with a power supply of 1.2 V (Figure 18).
A more recent publication by Genco et al.[105] describes the design of a multisensor device in which the SiMoT technology is further developed. In this study, a group of 47 patients was screened for pancreatic cancer precursors. Single molecules in cyst fluid and in blood plasma were detected using a portable 96-well bio-electronic sensor array, which can be applied directly in the point of care (Figure 19). The pancreatic cancer precursors are mucinous cysts diagnosed with a sensitivity of not more than 80% by existing cytopathological molecular analyses (e.g. KRAS gene mutation). The simultaneous detection of proteins related to the malignant transformation (such as MUC1 and CD55) is considered to be necessary for increasing the diagnostic accuracy. The proposed bio-electronic array based on SiMoT technology is able to detect nucleic acids and proteins with limits of detection down to one molecule and with <1% of false positive and false negative results.
The SiMoT-based bio-electronic array proposed by Genco et al.[105] is capable of detecting both nucleic acids and proteins. The device showed 96% diagnostic sensitivity and 100% diagnostic specificity. The developed SiMBiT bio-electronic platform prototype uses SiMoT technology for the EGOT sensor active layer and has a miniature reusable signal reader; data from the reader can be transmitted via a USB connection and processed with machine learning methods (Figure 20). Currently, this product can be considered to approach most closely the practical use as a POC device[105].
The possibility of using various approaches to the design of EGOFETs brings a certain degree of freedom to the design of multisensor systems based on them: instead of modifying the semiconductor layer, it is possible to use a multigate system with different recognition biolayers for analyte detection, as demonstrated by Parkula et al.[49] (Figure 21). The approach they used resulted in a lab-on-chip type device with a potential POC application and the possibility of measuring three samples simultaneously. The resulting multiplexable organic electronic lab-on-chip device provides a statistically valid and selective response for a microlitre sample volume within a minute.
The data presented above provide the conclusion that multisensor EGOFET technology is becoming increasingly commercialized: EGOFET-based portable devices are being designed and, as expected of EGOFET architecture, they can detect exceptionally low analyte concentrations outside a laboratory.
7. Aptamers based on nucleic acids as recognition elements
Nucleic acid-based aptamers (below referred to as aptamers) are structured oligonucleotides capable of high-affinity binding to a specific target. An example of DNA aptamer for the Escherichia coli tetracycline repressor is depicted in Figure 22. The aptamer has a complementary surface, which provides a set of non-covalent interactions with the protein, with the dissociation constant of the complex being 5.6 nM. Aptamers can be based on DNA, RNA and artificial nucleic acids. Non-natural nucleotides expand the scarce alphabet of natural nucleic acids, providing an enormous number of possible recognition elements[108-110]. The simplest classification of artificial nucleotides includes the following classes:
1. Modified riboses or deoxyriboses, including 2'-F, 2'-NH2, 2'-OМе and 4'-SH modifications, cyclic (locked) and acyclic (unlocked) L-ribose, L-deoxyribose and other sugars. These modifications are used, first of all, to increase the nuclease resistance of natural nucleic acids. Nevertheless, several examples of increased affinity to targets have also been reported[111, 112].
2. Modified phosphates, including those containing OCH3, S, SH, CH3, guanidine-like and other groups. These modifications are also meant to increase the nuclease stability of natural nucleic acids; in addition, they can affect the conformations of nucleic acids due to changes in the electrostatic repulsion between the phosphates of the sugar phosphate backbone, thus increasing the affinity of aptamers to the target[113, 114].
3. Modified heterocycles:
— a broad range of natural nucleotides with new substituents in the ring: amino acids, azides, peptides, aromatic hydrocarbons, aliphatic groups, biotin, fluorophores, etc. Substituents do not affect the hydrogen bonds between nucleotides, but provide a new surface for target recognition. This is the most popular method for increasing the affinity of aptamers[115, 116];
— new heterocycles mimicking the hydrogen bonds of natural nucleotides, for example, dDs – dPx base pairs [Ds: 7-(2-thienyl)-imidazo[4,5-b]pyridine, Px: 2-nitro-4-propynyl-pyrrole] and dZ – dP base pairs [dZ: 6-amino-5-nitro-3-(10-β-D-20-deoxyribofuranosyl)-2(1H)-pyridone, dP: 2-amino-8-(10-β-D-20-deoxyribofuranosyl)imid-azo[1,2-a]-1,3,5-triazin-4(8H)-one], phenoxazines, etc. New heterocycles are used to increase the affinity and to provide a ligand-dependent change in the fluorescence[117, 118].
4. Conjugation with other molecules such as lipids, proteins, peptides, drugs, polymers and nanoparticles[119, 120].
The selection procedure called SELEX (systematic evolution of ligands by exponential enrichment) has been significantly changed since the first use in 1990[121, 122]. The first SELEX procedures required 10 – 15 cycles of affinity selection of aptamers from DNA library with random sequences (below referred to as library). The aptamers were bound to the immobilized target and separated from the unbound oligonucleotide fraction. Amplification of aptamer-enriched libraries by polymerase chain reaction (PCR) was conducted between the cycles. The libraries composed by several SELEX cycles were cloned into bacterial plasmids and replicated in Escherichia coli culture. Then the plasmids were purified and sequenced. Single aptamers were chemically synthesized and evaluated for their affinity to the target. In the 1990s, the procedure was fairly labour-intensive and expensive.
To date, dozens of SELEX variants have been proposed that minimize the number of selection stages and simplify the stages. Chemically modified combinatorial libraries have an almost unlimited variety of sequences for selecting new aptamers. When microfluidic devices are used, the efficiency of aptamer selection increases owing to higher probability of interaction with the target due to the large immobilized target surface area accessible to a solution of a nucleic acid library being pumped; the average number of selection cycles is thus reduced to 3 – 5. Owing to new-generation methods of sequencing, this can be performed after PCR amplification of aptamer-containing libraries without DNA cloning and bacterial culturing. A few techniques have been proposed to optimize the selection of aptamers for small molecules, proteins, viruses, living cells and even living organisms[20, 123]. The selectivity of aptamers towards related targets can be programmed by introducing these targets into the selection cycles[124]. Currently, SELEX is a high-throughput routine procedure, which is quite competitive with preparation of antibodies and has a strictly controllable stages in vitro. New technologies have significantly reduced the cost of aptamer development and synthesis; this accounts for the great interest in their use as drugs and recognition elements of biosensors.
An aptamer-based drug (pegaptanib) was approved for the treatment of age-related macular degeneration, while a dozen of products are under various phases of clinical trials[121, 125]. An interesting line of research is the preparation of functional materials based on aptamers. The design of these materials makes use of the unique possibilities of site-specific modification and conjugation of aptamers with other molecules. Aptamers are widely used as recognition elements in drug delivery systems; in this case, aptamers are conjugated with chemotherapeutic drugs, polymers, proteins, short interfering RNAs, etc[125-129]. One more actively developing trend is related to aptamer-functionalized materials for chemo-and biosensorics (see below).
8. Multiplex sensors based on aptamers
Aptamer-based sensors (aptasensors) developed for various targets possess remarkably low limits of detection and broad analytical ranges of determination. In recent years, several reviews have been published comparing different approaches and analytical methods for aptamer-based detection of small molecules[11, 130-132], proteins[133, 134], viruses[134-136] and living cells[130, 137]. Electrochemical and optical aptasensors are most popular, due to the ultralow limits of analyte detection, high accuracy and good reproducibility of the procedures, and also the possibility of miniaturization of equipment to enable application in hospitals. For example, a few virions of SARS-CoV-2 (coronavirus 2 associated with severe acute respiratory syndrome) in a sample can be detected by surface-enhanced Raman spectroscopy (SERS)[136, 138]. The lowest limit of detection of microRNA-21 (1.35 × 10–18 М) was attained using pulse voltammetry[139], while the EpCAM protein (epithelial cell adhesion molecule) was detected in a concentration of 10–19 mol L–1 by an electrochemical aptasensor equipped with quantum dots[140].
A wide variety of aptasensors have been described to date. In this review, we consider the multiplex aptasensors and discuss modern procedures for simultaneous detection of several analytes in complex mixtures of variable composition, which implies a very high specificity towards the target. The bibliography includes publications of the last five years that reflect the key achievements in this area.
Aptasensors can be classified in terms of the principle of generation of the analytical signal. In this case, the following processes can be distinguished (Figure 23):
— direct determination: the sensor identifies the binary complex of the aptamer with its target;
— sandwich-like assay: assembly of ternary complexes; in this case, the aptamer;
— target binary complex is labelled with a third component generating the analytical signal;
— competitive assay: aptamer dissociates from some complex in the presence of a target; the analytical signal is caused by dissociation of this complex.
8.1. Direct determination
Direct determination is the simplest and the most reliable approach, which, however, requires very sensitive methods to provide very low limits of detection.
Methods based on the Förster resonance energy transfer (FRET) are particularly popular in aptasensors, as they can be modified with fluorophores and their quenchers. If these two subjects approach each other upon a change in the aptamer conformation, the fluorescence of the fluorophore decreases by several orders of magnitude. The conformational switches can be easily evaluated by FRET. The assay multiplexity is limited by the number of fluorophores with non-overlapping spectra. Graphene oxide is an effective quencher for many fluorophores. Youn et al.[21] proposed to use fluorophore-labelled aptamers, which are adsorbed on graphene oxide in the absence of appropriate targets (such as small molecule antibiotics, sulfadimethoxine, kanamycin and ampicillin), but are dissolved in the presence of targets. The LoDs were in the range of 5.5 – 6.7 nM, which is several times lower than the maximum permissible concentrations for foodstuffs. The analysis was performed in complex mixtures such as milk. This procedure is not suitable for real-time measurements.
Dielectrophoresis is based on the charge-dependent movement of micro- and nanoparticles in a non-uniform electric field. The surface charges of microparticles were measured using a microdielectrophoretic device with optical detection of the microparticle trajectory. The microparticles were functionalized with aptamers for Hg2+ and Ag+ cations, which changed the charge of species. The LoD values were 5.6 aM for Hg2+ and 115 fM for Ag+ when a multiplex sensor was used[22]. This procedure is not suitable for real-time measurements. Electrically neutral analytes cannot be determined by this method.
A portable device for electrochemical impedance spectroscopy was used to determine hormones, cortisol and neuropeptide Y, in a human sweat sample. Using non-Faradaic impedance spectroscopy, it is possible to estimate the interactions occurring at the electrode/electrolyte interface and to determine binary complexes without the use of redox probes or labels. The electrodes of the portable device were functionalized with thiol-modified aptamers. The aptasensor detected cortisol and neuropeptide Y with LoDs of 2.8 nM and 230 fM, respectively[141]. The sensor proved to be effective in the clinical validation of patient’s sweat samples and is potentially suitable for real-time probing. The sensor multiplexity can be increased by combining several devices.
Jiang et al.[23] considered an example of electrochemical detection of SARS-CoV-2 (Figure 24)[3]. The aptamers were immobilized on gold nanoparticles, which were electroplated on screen-printed electrodes. The electrochemical properties of the electrodes depended on the concentrations of the target proteins, that is SARS-CoV-2 spike (S) or nucleocapsid (N) protein. Excellent LoD values equal to 2 aM for the spike protein and 20 aM for the nucleocapsid protein were established (cyclic voltammetry was used). It is of interest that, compared to aptasensors, sensors with immobilized antibodies provided 48 and 1280 times higher LoDs, respectively. The developed sensors proved to be effective for clinical validation of nasopharyngeal swab samples of patients and are potentially suitable for real-time detection. The multiplexity of the sensor can be increased by combining a few devices[23].
One more example of highly sensitive detection is a multiplex aptasensor based on SERS for the detection of four viruses: SARS-CoV-2, influenza A, respiratory syncytial virus and adenovirus[142]. The sensor included four zones with gold nanocolumns, which were functionalized with thiol-modified aptamers with fluorophores. The fluorophores were resonant Raman dyes providing high intensity of SERS spectra. Binding of viruses induced a change in the intensity of SERS spectra due to reorientation of dye molecules relative to the surface. The limits of the detection of the aptasensor (the number of viral particles in 1 mL) were 100 for SARS-CoV-2, 600 for influenza А, 70 for type 3 and 5 adenovirus and 30 000 for the respiratory syncytial virus A2[142]. The sensor can operate in dilute complex biological media. The sensor multiplexity can be increased by combining a few SERS zones in the sensor. The sensor is potentially suitable for real-time measurements.
8.2. Sandwich-like assays
Sandwich-like assays are, most often, highly specific, but are unsuitable for detection of small molecules, as they do not have a sufficiently large surface for the interaction with two recognition molecules. Proteins, viruses and cells are good targets for sandwich-like assays.
Saraf et al.[25] proposed a simple, portable, and convenient-to-use aptamer-based device for multiplexed detection of viral proteins and viruses. The microfluidic device has aptamer-modified zones to capture and concentrate specific viral proteins or viruses. The collected material is stained with aptamer-modified gold nanoparticles; then it is coated by silver, which provides a grey colour in the testing zone. The colour intensity depends on the concentration of the analyte, which opens up the possibility of quantitative determination of the viral load. The limit of detection of the Zika and chikungunya virus envelope proteins is only 1 pM in a buffer. The instrument operated in a complex mixture (undiluted blood), and LoD was 100 pM. The device is not suitable for real-time readout.
The most well-developed aptasensor platform is SOMAscan[143, 144]. Assays using this platform consist of several stages and intermediate manipulations and provide the detection of up to 7000 analytes in 50 – 100 mL samples of biological fluids[143, 144]. In the first stage of the analysis, fluorophore-labelled aptamers are attached to microparticles via a photocleavable linker (Figure 25а). The protein targets bind to aptamers (Figure 25b). Then the proteins are biotinylated using an enzyme (Figure 25с). Then linkers between the aptamers and microparticles are cleaved under the action of ultraviolet light (Figure 25d ). The released complexes bind to streptavidin-coated particles (Figure 25е, f ). Then aptamers are washed away, and fluorescence is measured quantitatively with a microchip with complementary DNA chains (Figure 25g,h). The fluorescence intensity is proportional to the amount of protein in the sample. A single experiment does not cover all necessary concentration ranges of proteins that can be quantitatively determined; therefore, three-fold dilution of biological fluids is proposed. The total dynamic range is up to 108, with typical LoD values being in the picomolar concentration range. The average deviation factor is <5%, which attests to the possibility of excellent scalability of the approach; however, for some analytes, the deviation factor may be ≥50%[143, 144]. The SOMAscan platform is unsuitable for real-time measurements.
8.3. Competitive assay
In the competitive assay, the aptamer is released from a low-affinity complex in the presence of the target to form a new, high-affinity complex. Dissociation of the low-affinity complex gives rise to an analytical signal. Usually, the initial complex is attached to some surface. It is also possible to use soluble systems, but they are not reusable and cannot be serve as flow sensors for real-time applications.
In one competitive approach, aptamers are stained with a dye for single-strand DNA in a microfluidic chip wells[26]. The complexes exhibit intense fluorescence, which decreases in the presence of targets: microcystin-LR, cylindrospermopsin, nodularin and anatoxin-A. The single-strand DNA-binding dye shows a low quantum yield when it is not complexed with DNA; therefore, the rearrangement of the complex can be easily detected. A portable device was manufactured using a common smartphone instead of a stationary fluorimeter. This approach is promising, as it does not restrict the choice of targets and ensures rather low LoDs (in the range of 1 – 3 nM), which corresponds to the maximum permissible concentrations of toxins in drinking water[26]. This assay is unsuitable for real-time measurements.
Resistive pulse sensing is used to measure the rate of translocation of single nanoparticles and gain quantitative information about the nanoparticle charge[21]. The nanoparticles are modified by anchoring DNA, which reversibly bound aptamers thus forming short double helices of DNA. These complexes were destroyed in the presence of aptamer targets that decrease the nanoparticle charge. Using resistive pulse sensing method, it was possible to distinguish between three targets due to the different sizes of nanoparticles modified with appropriate anchors. The limits of detection were not superior to those attained in the direct determination, but the assay specificity was high. Maugi et al.[145] detected a small-molecule antibiotic moxifloxacin and small-molecule anticancer drugs imatinib and irinotecan with LoD values in the micromolar range. This procedure can be supplemented by magnetic separation to provide sample purification and the possibility of nanoparticle reuse. Further increase in the number of targets is complicated by the presence of nanoparticles that appreciably differ in size. The assay is unsuitable for real-time measurements.
A similar competitive assay was developed for determination of whole viruses[146]. The nanostructured gold surface was functionalized with a thiol-modified oligonucleotide complementary to the aptamer, while two Raman labelled DNA aptamers were hybridized with capture DNA on the gold nanopopcorn substrate (Figure 26а). The 4-MBA internal standard was immobilized, together with DNA aptamers, on the gold nanopopcorn substrate. The recognition of the target protein induced a conformational change in the aptamer, resulting in a decrease in the intensity of the Raman scattering signal (Figure 26b,c). Using the aptasensor, the authors were able to detect SARS-CoV-2 [LoD of 0.78 plaque-forming units (PFU) in 1 mL] and influenza A virus А [LoD of 0.62 hemagglutination units (HAU) in 1 mL][146]. By using new dyes, the number of targets can be slightly increased. This assay is potentially suitable for real-time measurements.
Analysis of the latest trends in the studies of aptamers showed that they are applicable as recognition elements in multiplex sensors. The affinity and specificity of aptamers provide very low LoDs in the determination of targets in complex mixtures. Several types of aptasensors are applicable for real-time determination, which is necessary, for example, for flow sensors used for environmental monitoring (Table 2). Most of new aptasensors envisage direct determination of binary aptamer —target complexes, and this ensures one-stage determination of the target. In these aptasensors, ultralow LoDs are due to sensitive analytical methods such as electrochemical measurements or SERS spectroscopy. Further research and development in this field are aimed at miniaturization and portability of equipment for medical care in field conditions.
9. Prerequisites for the increase in efficiency of multisensors based on electrolyte-gated organic field-effect transistors modified with aptamers
To date, various types of multisensor devices with aptamers as recognition elements have been described and are successfully used. Indeed, aptamers were used as recognition elements for the simultaneous detection of several bioanalytes by various sensors (including sensors with colorimetric[147] and electrochemical[148-150] measurement of the analytical signal and field-effect transistor-based sensors[15]) (Figure 27).
The main advantage of these devices over monosensors is the shorter time of analysis, simpler sample preparation procedure and potential applicability of these systems for industrial manufacturing. EGOFET-based devices are perfect candidates to serve as basic elements of multisensors for bioanalytes owing to signal amplification, low operating voltage and simple fabrication.
Aptamers have attracted attention of researchers, as they possess a number of advantages over antibodies such as specificity, high affinity to the analyte and also chemical, thermal and conformational stability. These antibody analogues easily undergo reversible folding when bind to the target molecules[38, 152] and, unlike antibodies, they can be obtained in vitro for any analyte. These advantages make aptamers much more convenient materials for production of recognition layers than antibodies.
Thus, multisensing EGOFET devices can be perfectly combined with aptamer-based recognition layers, and this has been repeatedly confirmed by modern designed products. For example, Poimanova et al.[85] carried out fast and selective quantitative detection of influenza virus by aptamer-based EGOFET sensor. The multisensor cell (Figure 28) is simply fabricated and allows simultaneous conduction of several measurements, which markedly simplifies the analysis. It was shown that the thinner the semiconductor layer the higher the biosensor sensitivity.
Currently, the scope of applicability of aptamers in EGOFET devices is increasingly expanding: as the number of available aptamers increases, it becomes possible to identify early markers of dangerous diseases including infections, inflammation and malignant neoplasms. Solodka et al.[83] showed the possibility of determination of interleukin IL-6, which is a multifunctional cytokine. The quantitative determination of IL-6 is of prime importance in the early stages of inflammation and in chronic diseases, since conventional methods are expensive, time-consuming and usually based on the use of fluorescent or radioactive labels. The proposed biosensor is based on EGOFET in which the gate is coated with monoclonal antibodies or peptide aptamers. Both types of functionalization provide LоDs equal to several tens of picomoles in 1 litre.
White et al.[153] used a microfluidic system of aptasensors for the detection of ricin (Figure 29) with LoD value of 30 pM. The assay was carried out in the flow mode, which made it possible to carry out measurements within a few minutes, because no pretreatment, enzymatic reaction or washing was required. The simplicity of manufacturing, multiplexity and simple operation of this device were noted.
EGOFET-based sensors demonstrated promising results in small-size, inexpensive and fast operating devices for the detection of various analytes on a real time basis. The electrical signals transmitted by electrolyte – insulator – semiconductor type devices can be recorded rather easily by standard instruments, and commercialization of technologies that can be integrated into microfluidic systems has recently received particular attention[154].
Theoretical models are being developed to describe the operation of aptamer-based sensors. Araujo-Rocha et al.[37] proposed a sensor based on DNA immobilized on the surface of gold. This sensor makes use of conformational switching, which changes the concentration of ions near the gold surface. This effect may give rise to label-free transduction, which may be used in a future EGOFET-based biosensor. In a theoretical study by Massey and Prakash[61], aptamers were also used to develop a theoretical description of EGOFET operation.
A number of publications address the development of an aptasensor-based EGOFET platform for the determination of various biomarkers related to the Parkinson’s disease[16, 81, 94]. The aptasensor with a linear range from 100 to 10 mg L–1 depicted in Figure 30 showed a LoD of 10 fg L–1 for the α-synuclein (αSyn) monomer in saliva samples[81].
Thus, the use of aptamers appears to be a facile and convenient method for modifying the recognition layer. Aptamer-based sensors are more stable to the environment and little affected by external conditions. These features make aptamers promising materials for the design of recognition layers to detect various markers over a wide range of concentrations. Currently, active research is carried out to design biosensors using aptamers as recognition layers based on EGOFET architecture and to develop theoretical descriptions of aptamer applications in sensor devices.
10. Conclusion
The past decade witnessed significant progress in multi-target biological analysis involving simultaneous detection of one or more analytes based on electronic and optical methods. Electrolyte-gated organic transistors possess exceptionally high sensitivity, which is combined with selectivity, a broad range of detectable analytes, a broad range of analyte concentrations and energy efficiency; they are easy to manufacture and use, smoothly operate even in complex biological materials such as blood or saliva. The above benefits account for numerous practical applications of these systems as biological sensors directly at points of medical care.
Currently scientists together with engineers develop various approaches to increasing the performance of these devices. There appear prototypes with several electrodes, potentiometric detection method (a reference electrode is used together with the gate electrode), conceptually new procedures of analysis using EGOFET architecture, which open up the possibility of single molecule detection (SiMoT technology). Studies of the theoretical mechanisms that form the basis for recognition of various biomarkers using EGOTs, including charge transfer mechanisms and enhancement of sensor response, are being continued. Quite a few publications are devoted to the development of approaches to the design of recognition layers as parts of EGOTs. Both semiconducting layers and the gate electrode are modified with antibodies and aptamers. Various architectures of electrolyte-gated transistors and methods for signal recording and analysis are selected. The design of demonstration prototypes and measuring devices capable of operation outside a laboratory involves the development of analogue electronic circuits for control of their operation, output signal transduction and multiplexing adapted to the sensors. These solutions serve for signal conversion to a form suitable for the subsequent digitization and integration into data collection devices performed both using separate electronic components and as integrated circuits.
Furthermore, the search for the design of a multisensor able to determine targets of various nature with equal efficiency is being actively carried out. Aptamers represent a promising recognition element for the design of such sensors, while a microfluidic cell with a set of functionalized EGOTs for different analytes mounted on one substrate can solve the problem of highly sensitive determination of a wide range of toxins and pathogens in liquid media. This possibility appears owing to combination of miniature size of the cell, easy use, operation in environments with high ionic strength (contaminated water, biological liquids) and fast selective electrical response to the analyte.
11. List of abbreviations and symbols
ADA — anti-drug antibody,
CMOS — complementary metal–oxide semiconductor,
EDL — electric double layer,
EG-CNTFET — electrolyte-gated carbon nanotube field-effect transistor,
EGOFET — electrolyte-gated organic field-effective transistor,
EGOT — electrolyte-gated organic transistor,
FRET — Förster resonance energy transfer,
GFP — green fluorescent protein,
HAR — hemagglutination reaction,
ISFET — ion-selective field effect transistor,
LoD — limit of detection,
NF — neurofilament,
OECT — organic electrochemical transistor,
OEGFET — organic electrolyte-gated field effect transistor,
OFET — organic field-effect transistor,
OTFT — organic thin film transistor,
PBS — phosphate-buffered saline,
PCR — polymerase chain reaction,
PFU — plaque-forming units,
p(h2T-TT) — poly(2-(3,3'-bis(2-(2-(2-methoxyethoxy) ethoxy)ethoxy)-[2,2'-bithiophen]-5-yl)thieno[3,2-b]thiophene,
pBTTT-C14 — poly[2,5-bis(3-tetradecylthiophen-2-yl)-thieno[3,2-b]thiophene],
P3HT — poly(3-hexylthiophene-2,5-diyl),
p3CPT — poly[3-(5-carboxypentyl)thiophene-2,5-diyl],
PEDOT : PSS — poly(3,4-ethylenedioxythiophene) complex with polystyrene sulfonate,
РОС — point-of-care,
SAM — self-assembled monolayer,
SELEX — systematic evolution of ligands by exponential enrichment,
SERS — surface-enhanced Raman spectroscopy,
SiMBiT —single molecule bio-electronic technique,
SiMoT — single molecule with a large transistor,
SPR — surface plasmon resonance,
IDS — drain – source current,
VDS — drain – source voltage,
VGS — gate – source voltage,
VG — gate voltage.
Acknowledgements
This review was written within the State Assignment on the subject FFSM-2022-0001 (Sections 2, 3, 4, 5) and with the financial support of the Russian Science Foundation (Project No 23-73-00103, Sections 6, 7, 8, 9).
References
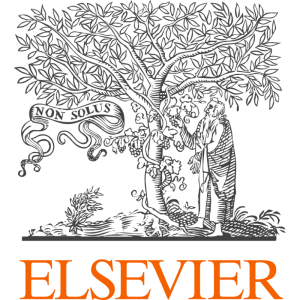
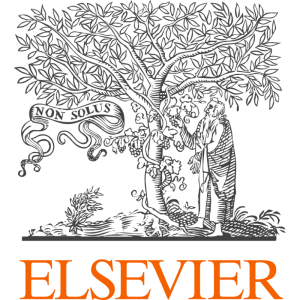
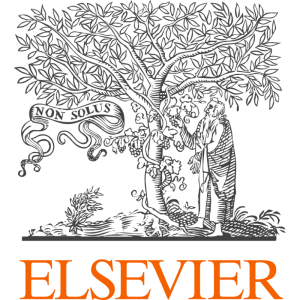
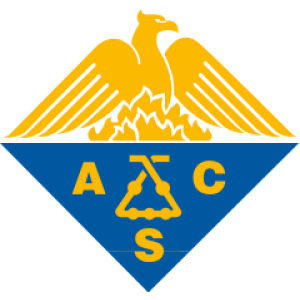
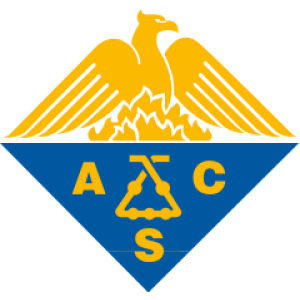
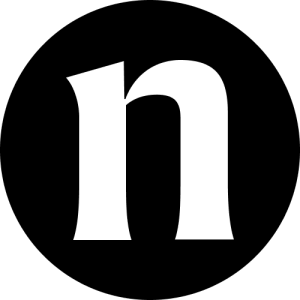
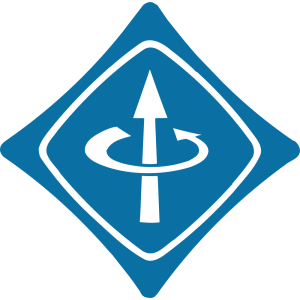
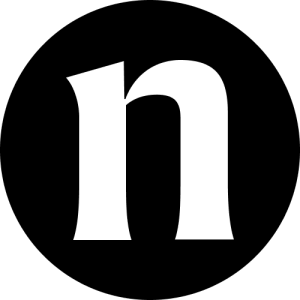
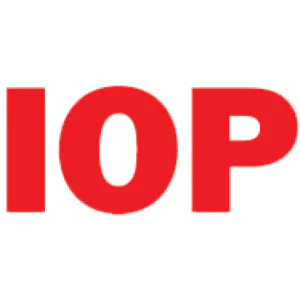
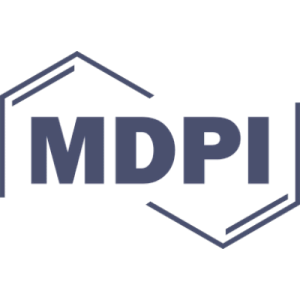
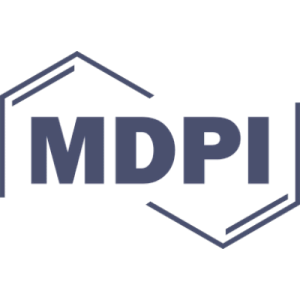
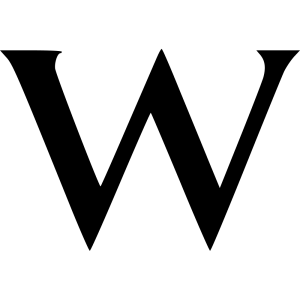
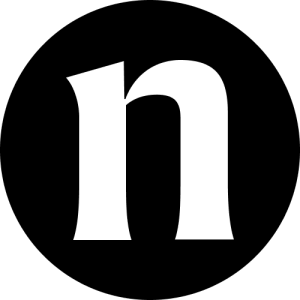
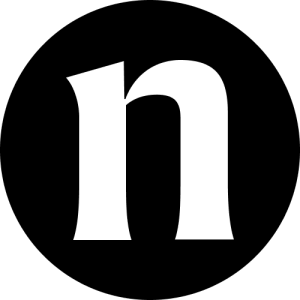
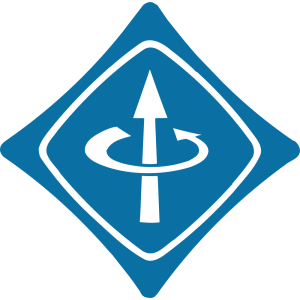
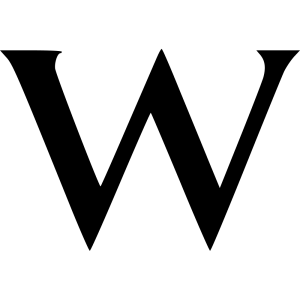
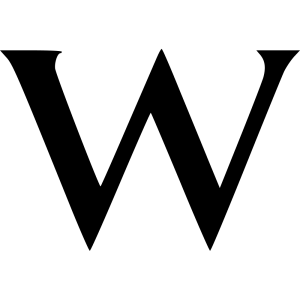
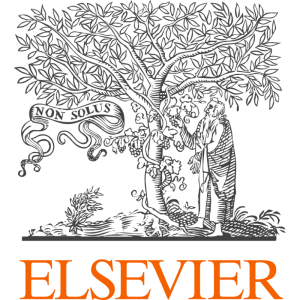
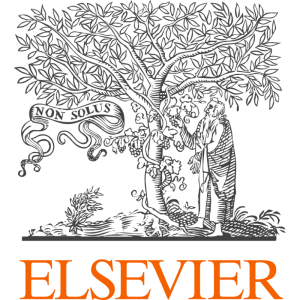
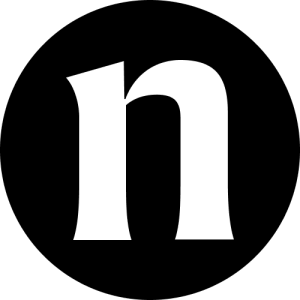
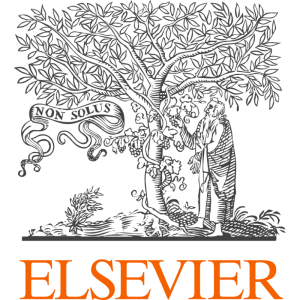
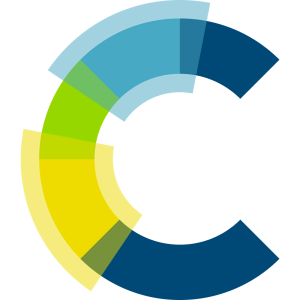
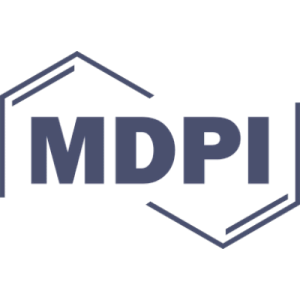
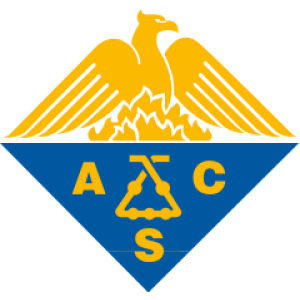
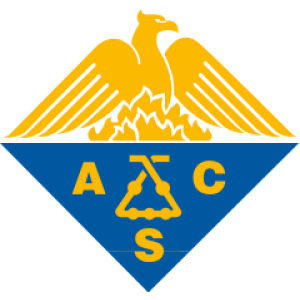
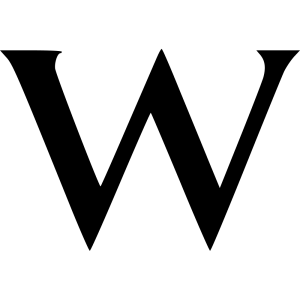
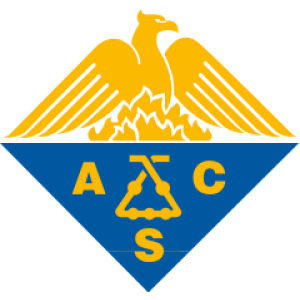
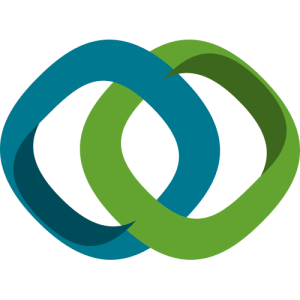
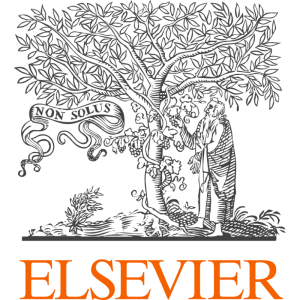
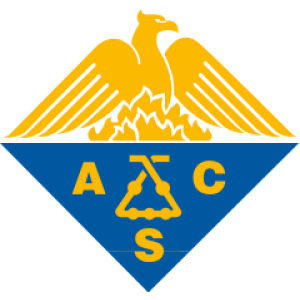
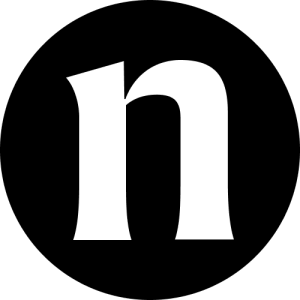
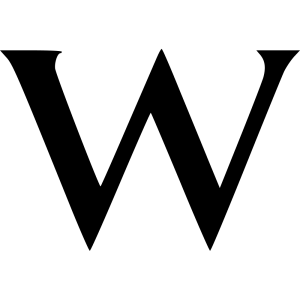
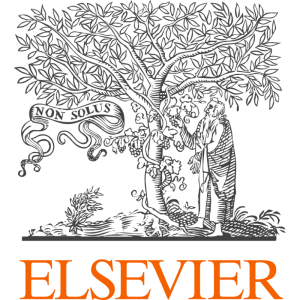
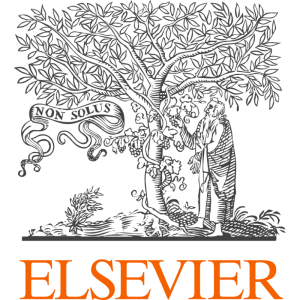
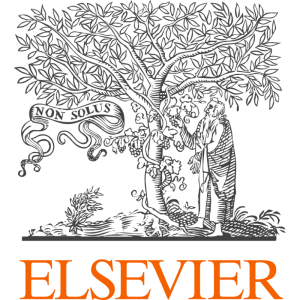
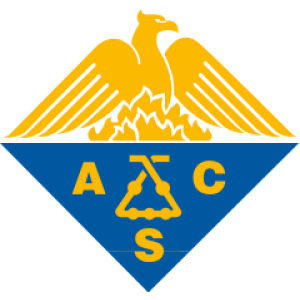
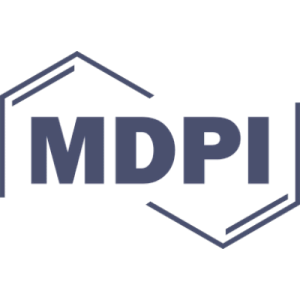
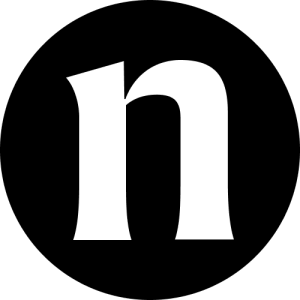
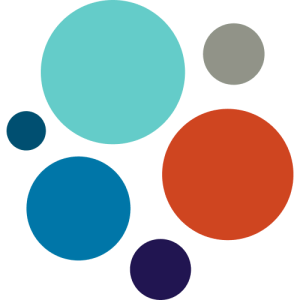
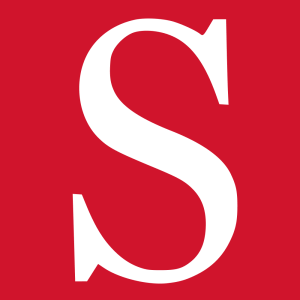
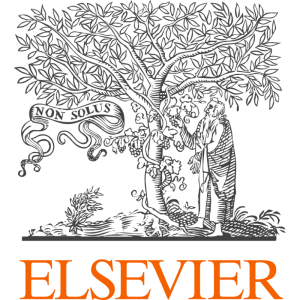
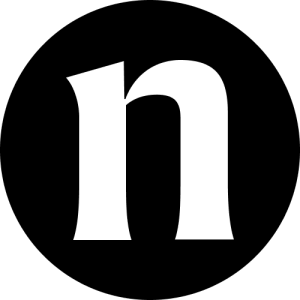
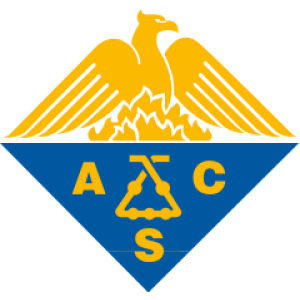
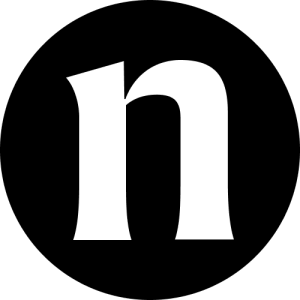
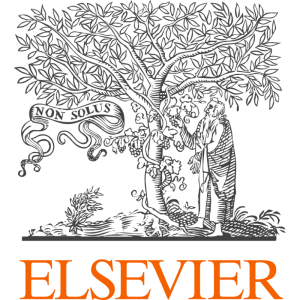
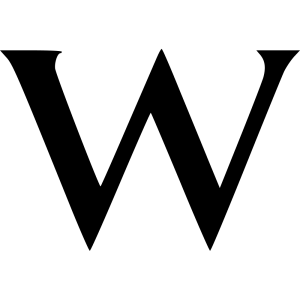
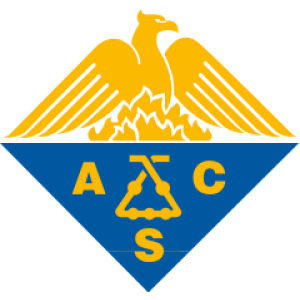
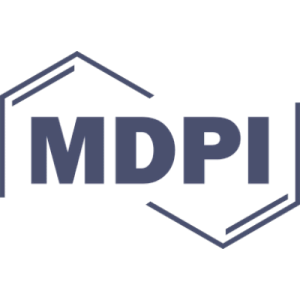
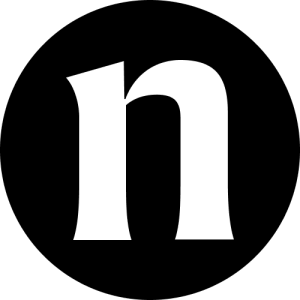
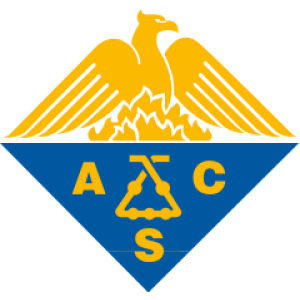
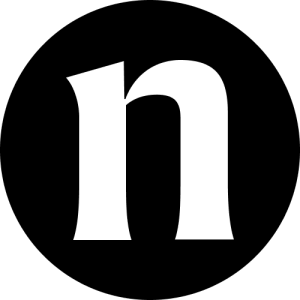
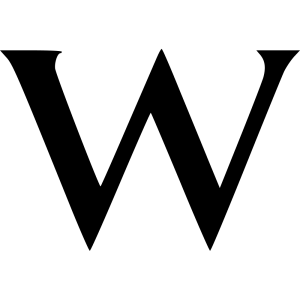
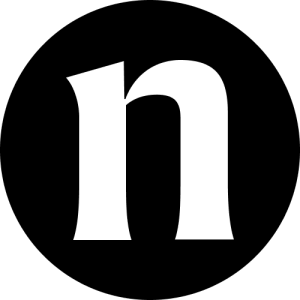
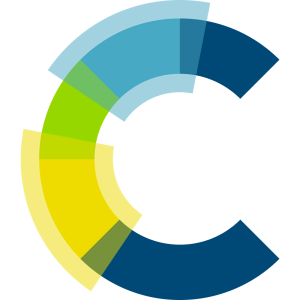
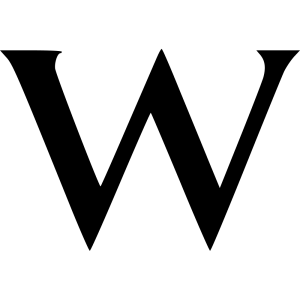
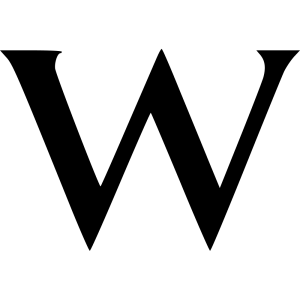
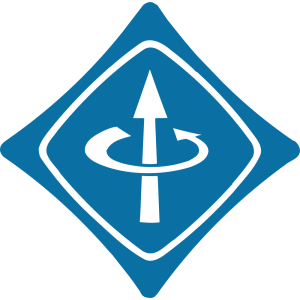
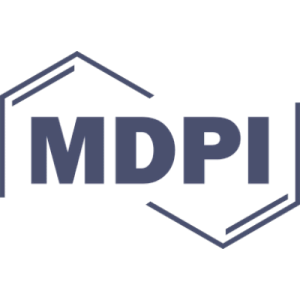
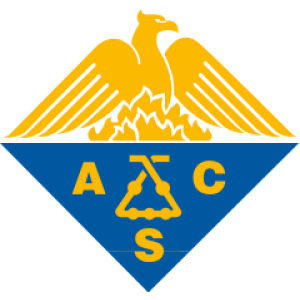
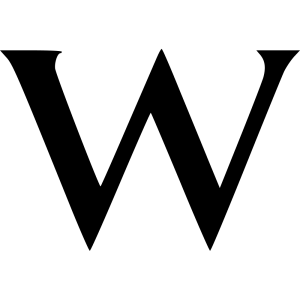
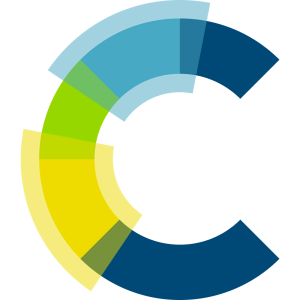
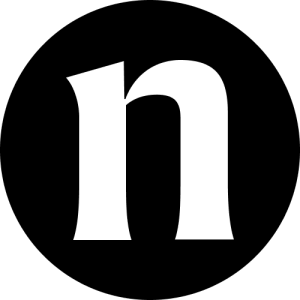
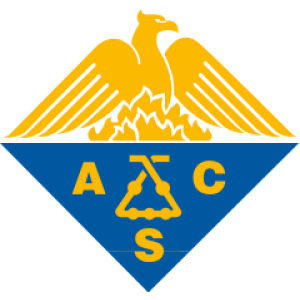
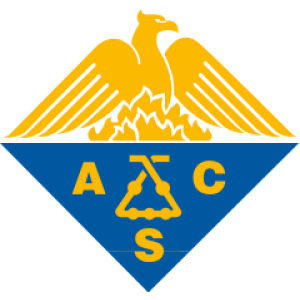
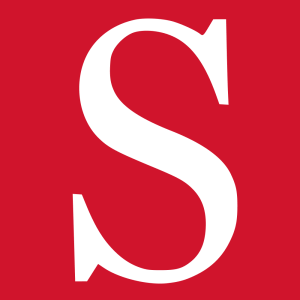
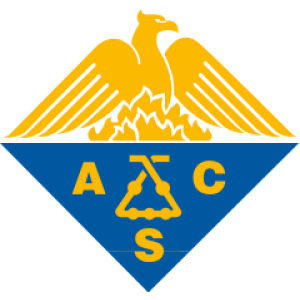
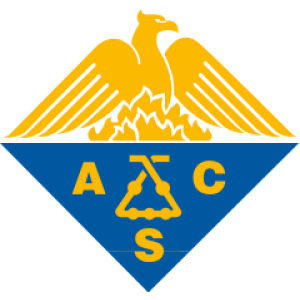
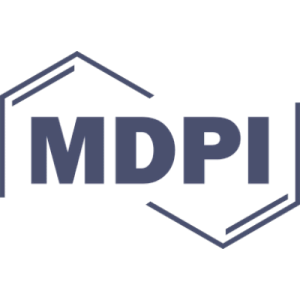
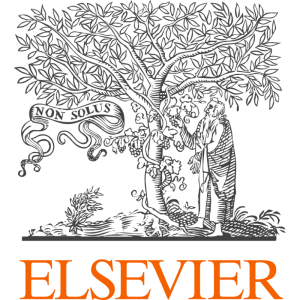
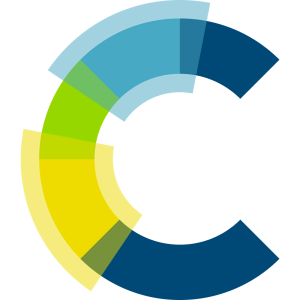
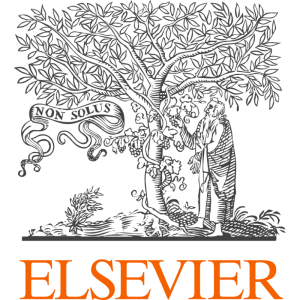
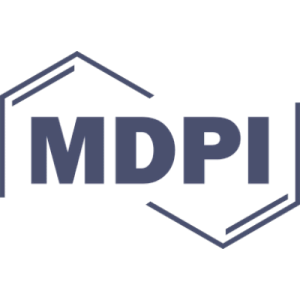
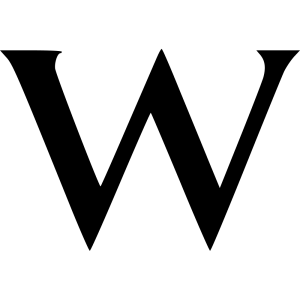
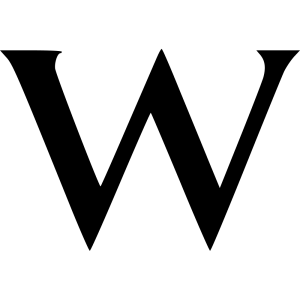
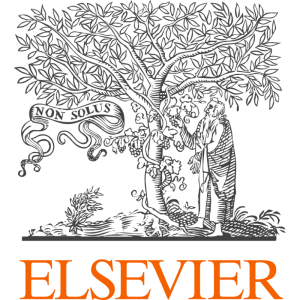
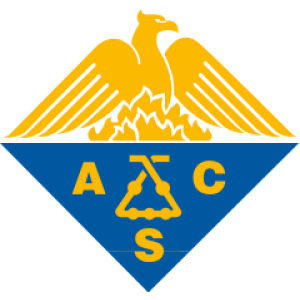
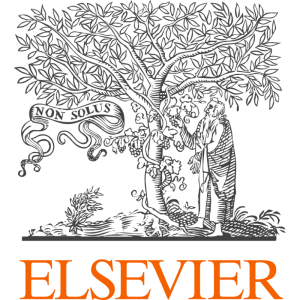
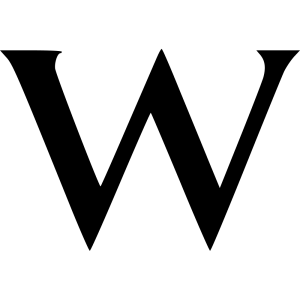
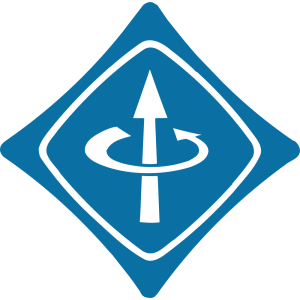
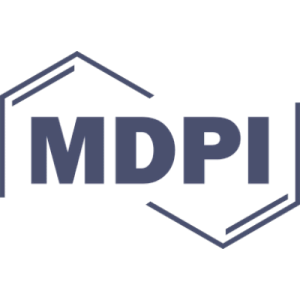
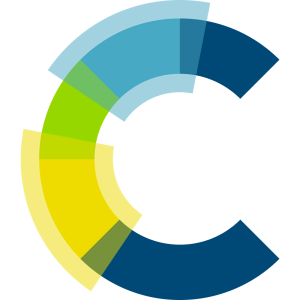
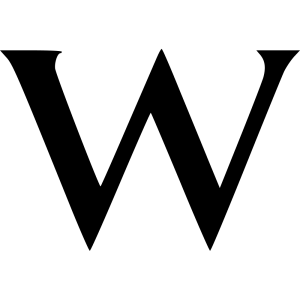
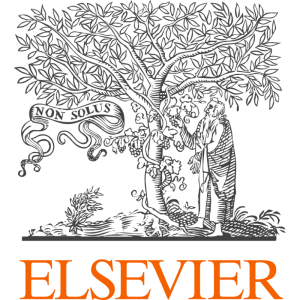
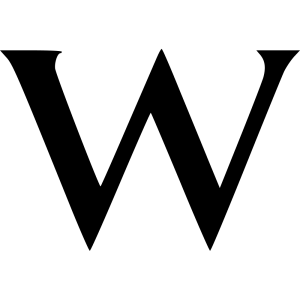
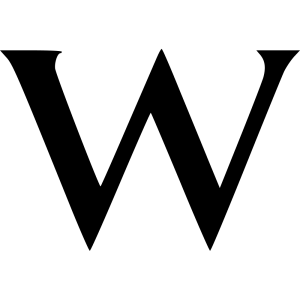
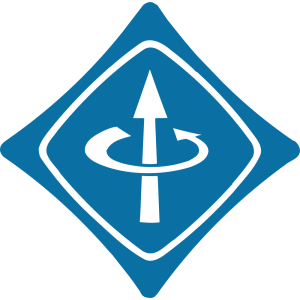
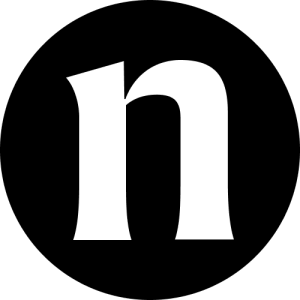
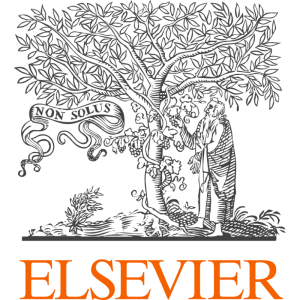
![Biorecognition Layer Based On Biotin-Containing [1]Benzothieno[3,2-b][1]benzothiophene Derivative for Biosensing by Electrolyte-Gated Organic Field-Effect Transistors](/storage/images/resized/iLiQsFqFaSEx6chlGQ5fbAwF6VYU3WWa08hkss0g_small_thumb.webp)
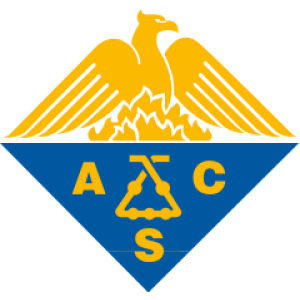
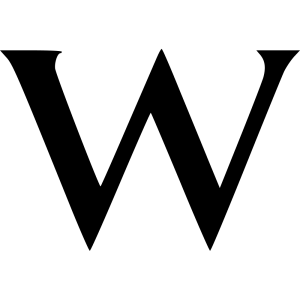
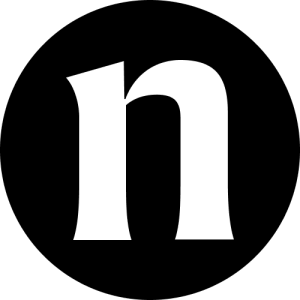
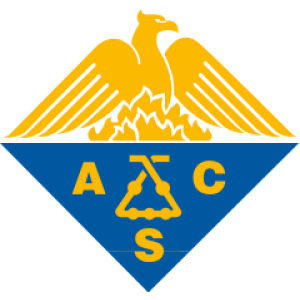
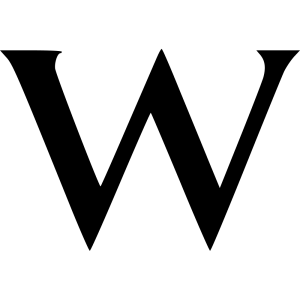
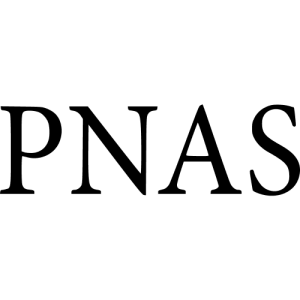
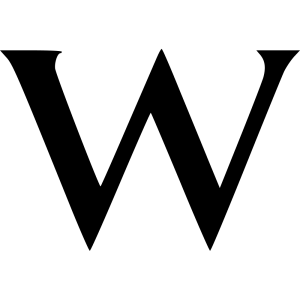
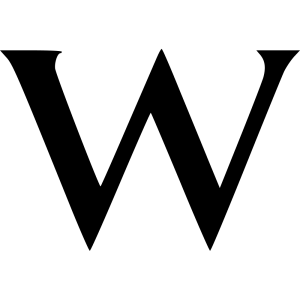
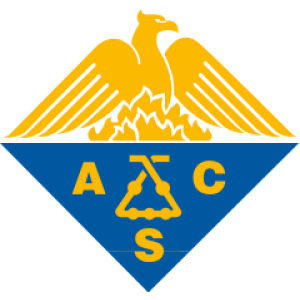
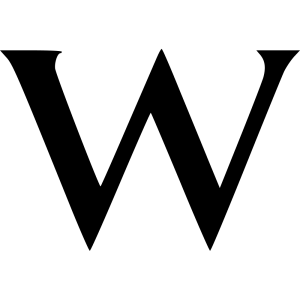
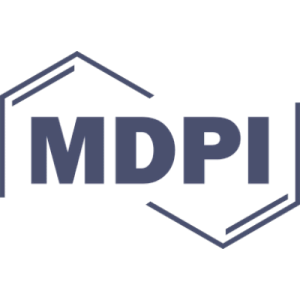
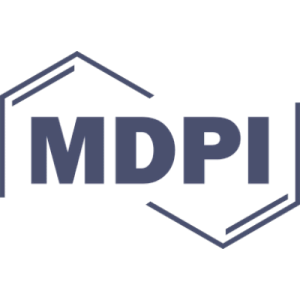
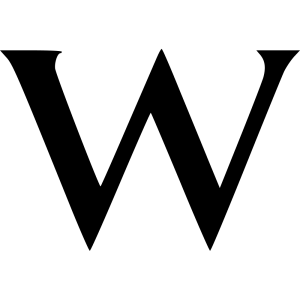
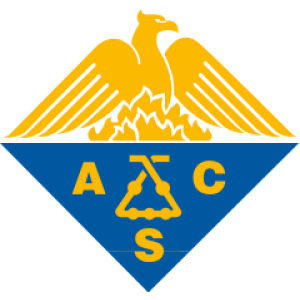
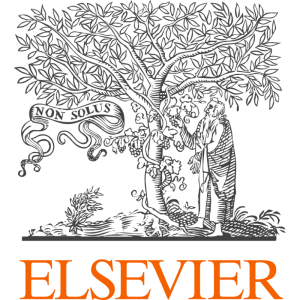
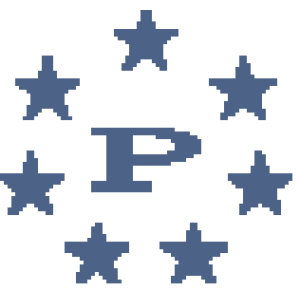
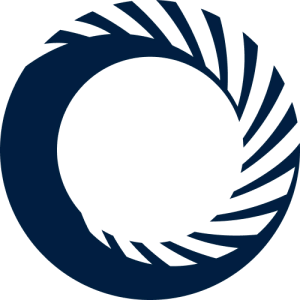
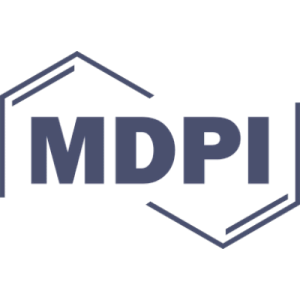
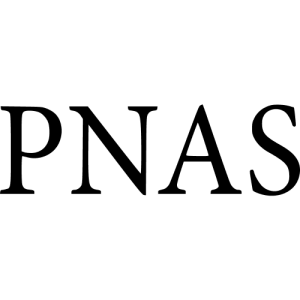
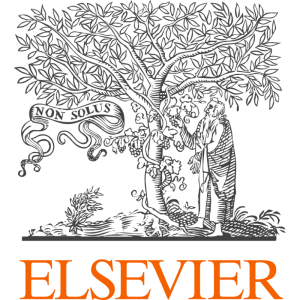
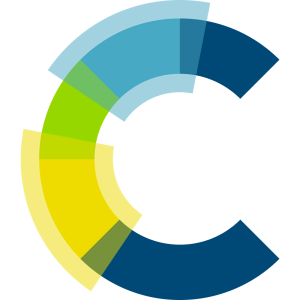
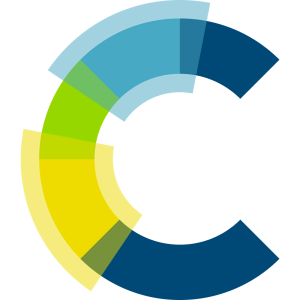
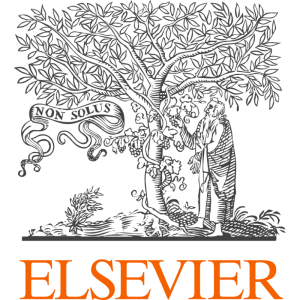
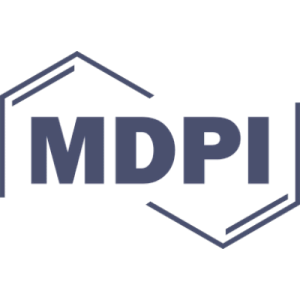
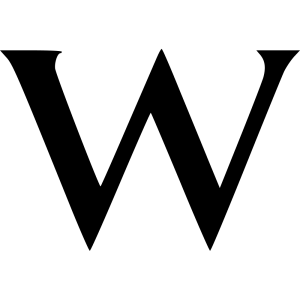
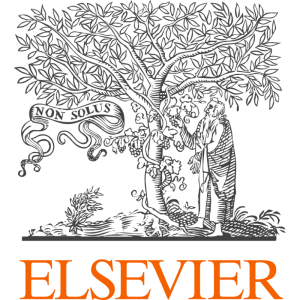
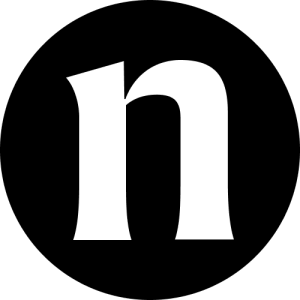
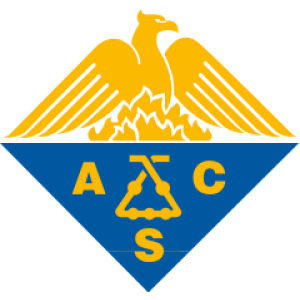
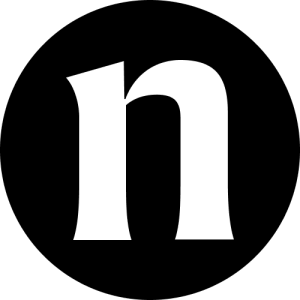
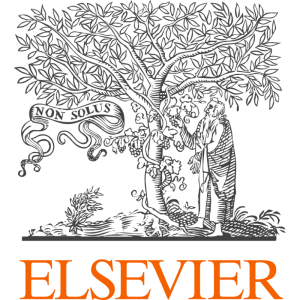
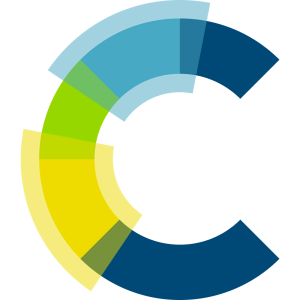
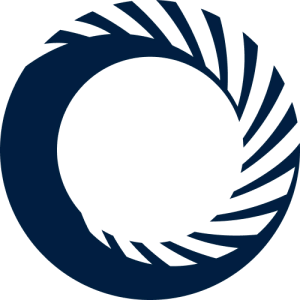
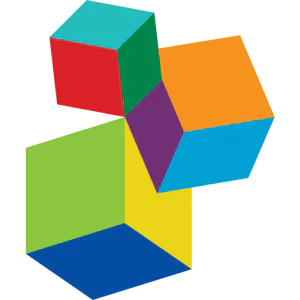
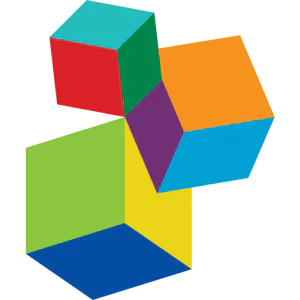
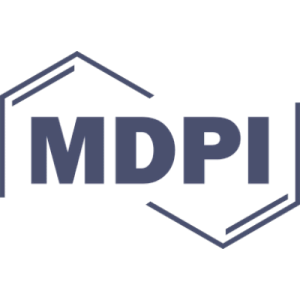
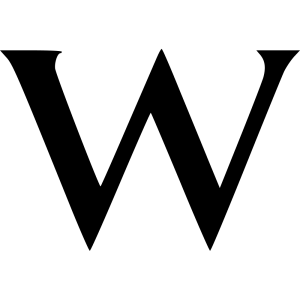
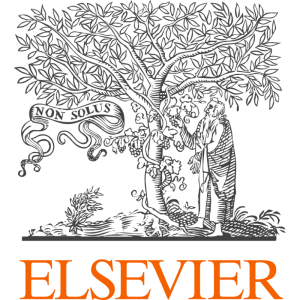
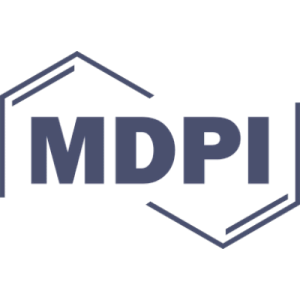
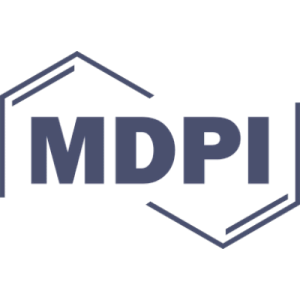
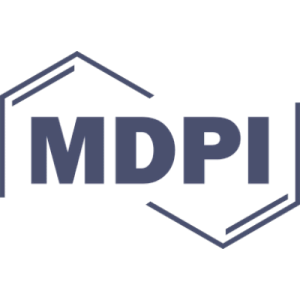
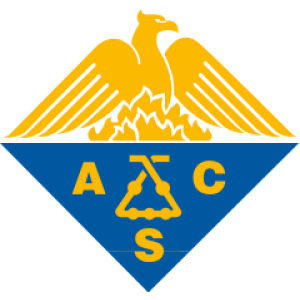
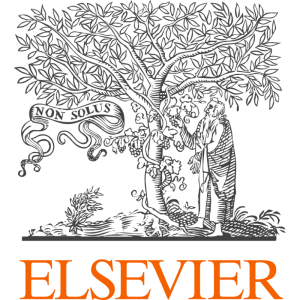
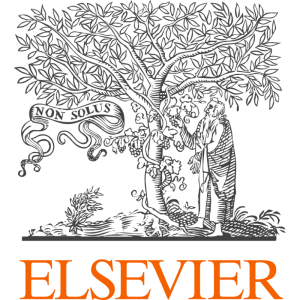
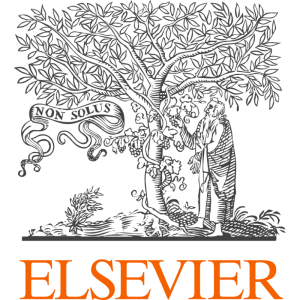
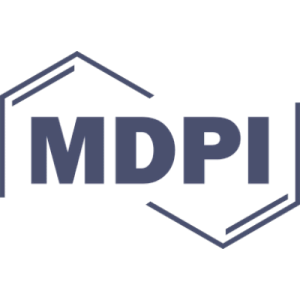
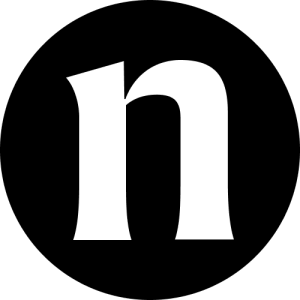
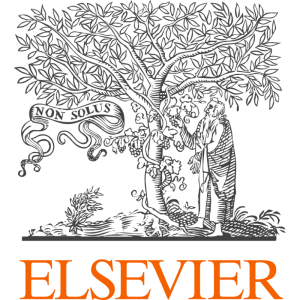
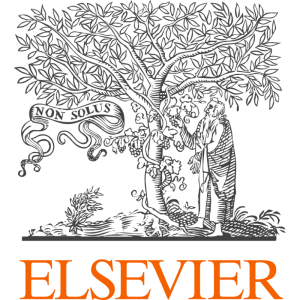
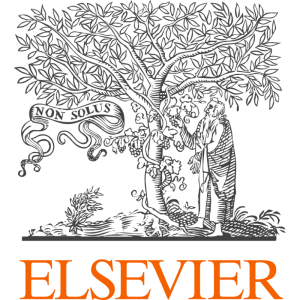
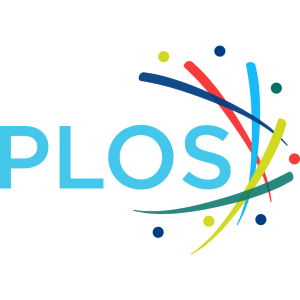
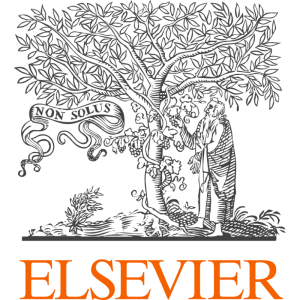
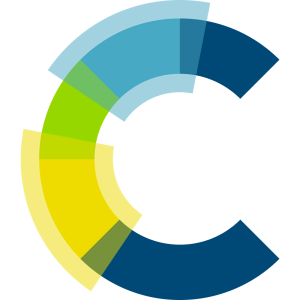
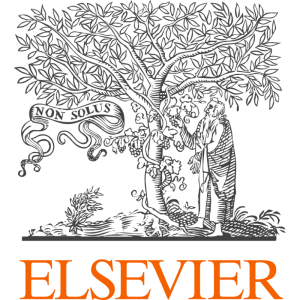
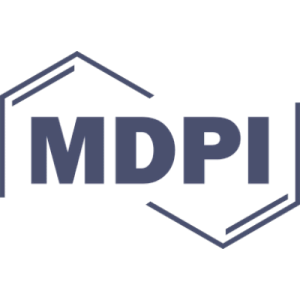
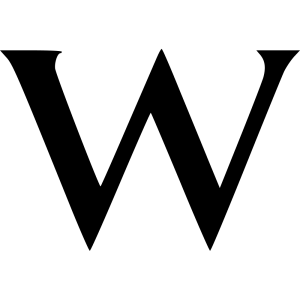
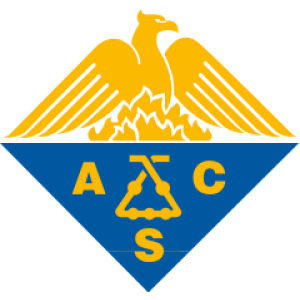
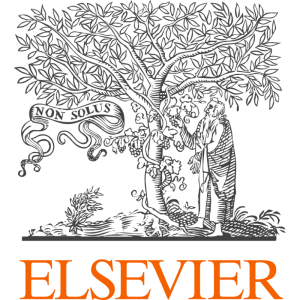