Keywords
Abstract
A review on the quantitative determination of aromatic monocarboxylic acids (AMCAs), intermediates in the metabolism of the proteinogenic amino acids phenylalanine and tyrosine, in body fluids is presented. The importance of these compounds as potential diagnostic low-molecular-weight biomarkers of some human diseases is described. The main methodical features of current practical solutions in the field of highly sensitive screening of AMCAs in body fluids using chromatography-mass spectrometry and other methods actively used in clinical laboratory analysis are considered. Special attention is paid to the prospects of developing portable tools for therapeutic monitoring of the compounds of interest in biological fluids.
The bibliography includes 108 references.
1. Introduction
Low-molecular-weight carboxyl-containing naturally occurring compounds, such as aromatic carboxylic acids, play an important role in detecting functional metabolic changes in humans and animals[1]. The content of these compounds in biological fluids can provide important information about the physiological and pathological status of different metabolic pathways and their interrelationships in a living body. In particular, for many years there has been active work aimed at a comprehensive study of the functional pathological changes associated with the biodegradation of aromatic amino acids such as phenylalanine and tyrosine. The results of extensive clinical and experimental studies have convincingly demonstrated that the levels and dynamics of changes in the intermediate metabolites of these amino acids — aromatic monocarboxylic acids (AMCAs) — in biological fluids are diagnostic indicators of metabolic disorders, often associated with inherited and acquired diseases, as well as markers that can be used to predict the development of infectious complications and outcome in the critically ill patients[2-4]. It should be noted that the introduction of small biomarkers into clinical practice is a trend in modern medicine. To date, many potential markers have been evaluated for possible use in the diagnosis of various diseases, but unfortunately, only a few have found application in clinical practice.
Monitoring the concentration of AMCAs (their anionic forms) is a real challenge because of their low content (ca. μmol L–1 or ng L–1), similar chemical structure and the complex composition of biological matrices, which practically excludes the direct determination of these substances. Sampling, storage and preparation of biomaterial for analysis are important because even small differences in these operations can affect the validity and reproducibility of the results[5].
Highly sensitive methods for the identification and quantification of AMCAs in the body’s biological fluids are the most important tools for successfully solving diagnostic problems, which, in combination with other tests, provides a more complete picture of the patient’s condition and lead to the most optimal treatment protocols. The development of portable sensor devices for rapid ‘bedside’ analysis is undoubtedly of interest to laboratory diagnostics, since early and timely diagnosis is the most important factor influencing the patient’s survival and recovery. Unfortunately, the high cost of developing a portable biomarker monitoring technology does not encourage investments in this area of research.
Current advances in the detection of functional metabolic changes in the body through the analysis of biological fluids are mainly associated with the application of chromatography-mass spectrometry (CMS)[6-11] and nuclear magnetic resonance (NMR) techniques. However, the high cost of these methods and the expendable materials needed to perform them, the time required for sample preparation and analysis, and the need for skilled specialists to operate and maintain the equipment all hinder their introduction into the routine clinical and laboratory practice. Not accidentally, the improvement and development of new techniques and devices for the detection and quantitative determination of clinically relevant phenylalanine and tyrosine metabolites has been and remains a relevant scientific task.
The aim of this review is to summarize the existing knowledge on the problem of quantitative determination of a number of AMCAs in biological body fluids, as well as to outline the prospects for development of this field in terms of the need to create portable tools of their rapid detection for in situ monitoring of the patient’s health status.
2. Phenylalanine and tyrosine metabolites as low-molecular-weight biomarkers of various human diseases
Metabolomics, an interdisciplinary field of science that comprehensively studies the metabolic profiles of living organisms, has developed rapidly in recent years. According to the current scientific understanding of the biochemical metabolic reactions of the human body, the determination of low-molecular-weight metabolites can help to identify many problems of the organism, outline a plan for the in-depth study and treatment. Thus, the content of some AMCAs in biological fluids appears to be an important diagnostic parameter for various inherited or acquired diseases and conditions. It has been established that the mechanism of the AMCA formation in the human body is directly related to changes in the biotransformation of proteinogenic aromatic amino acids, phenylalanine and tyrosine (Figure 1)[12].
In the liver of healthy individuals, only a small portion (∼10%) of phenylalanine is converted to 3-phenyllactic acid (PhLA) and phenylacetylglutamine[13]. This pathway of phenylalanine catabolism becomes predominant when the main pathway, the conversion of phenylalanine to tyrosine catalyzed by phenylalanine hydroxylase, is impaired. Deficiency or inborn absence of phenylalanine hydroxylase has been reported to result in transamination of L-phenylalanine with accumulation of a number of metabolites in body fluids, including 3-phenylpyruvic acid (PPA), L-PhLA (a reduction product of PPA) and 2-phenylacetic acid (PhAA), which is formed by decarboxylation and oxidation of PPA[13]. It is generally accepted that the L-form of PhLA is endogenous, whereas its D-form is of bacterial origin[14].
In vivo, the L-form of tyrosine is enzymatically degraded in a series of steps, the first and rate-limiting step being the enzymatic deamination and oxidation of L-tyrosine to 3-(4-hydroxyphenyl)-pyruvic acid (p-HPPA) under the action of tyrosine aminotransferase. The p-HPPA can be exposed to the enzyme 4-hydroxyphenylpyruvate dioxygenase to form homogentisic acid (HGA), decarboxylated to 2-(4-hydroxyphenyl)acetic acid (p-HPhAA) or alternatively reduced to 3-(4-hydroxyphenyl)-lactic acid (p-HPhLA). The D-form of p-HPhLA is a product of bacterial metabolism and is normally synthesized mainly by intestinal anaerobes, since the human gut microbiota is known to contain a large number of anaerobic bacteria, many of which have the metabolic potential to degrade a wide range of protein substrates[15]. The role of microbial metabolites and the microbial pathway of the AMCA formation in humans has been discussed in a number of publications[16-18]. In addition, the metabolic pathways of phenylalanine[19] and tyrosine[20] can be studied in detail using the open internet resource KEGG PATHWAY Database (https://www.genome.jp/kegg/pathway.html).
Microbial and endogenous metabolites of phenylalanine and tyrosine are found in a variety of biological samples, which can be classified as invasive (blood, cerebrospinal fluid, amniotic fluid, various tissues) and non-invasive (urine, faeces, saliva, sweat, hair) based on the need to penetrate the body’s natural external barriers to obtain them. Metabolites released from the intestine into the bloodstream may be conjugated in the liver by the neutralization and excreted in the urine. At the same time, it is likely that the most physiologically active forms are free, unconjugated metabolites, which can have an effect on the human body. No information on conjugation was found in the literature for metabolites of tyrosine and phenylalanine, such as PhLA, p-HPhAA, and p-HPhLA, which are diagnostically important in the intensive care. The absence of conjugates for PhLA, p-HPhAA, and p-HPhLA can be explained on the one hand by their higher polarity compared to, e.g., PhAA and consequently the lack of need to increase their polarity and solubility in blood. On the other hand, the explanation for the absence of conjugates may lie in the fact that there is a natural mechanism to remove the excess of these intermediates of microbial metabolism — their biotransformation into final products in the intestine involving normal gut microbiota. It is therefore no wonder that the highest levels of PhLA, p-HPhAA, and p-HPhLA are found in critically ill patients with sepsis, where gut dysbiosis is characterized by a pronounced depletion of the species composition of the normal microbiota, i.e. the natural mechanism of the biotransformation of these metabolites into final products is impossible[21, 22]. It should be noted that a large number of biomarkers of sepsis have been described, probably due to the very complex pathophysiology of this disease[23]. In particular, information on the ability of sepsis-associated bacteria to produce aromatic monocarboxylic acids (PhLA, p-HPhAA, and p-HPhLA) seems to provide a strong argument that their elevated levels in patient serum are closely correlated with sepsis severity and outcome and are due to microbial degradation of phenylalanine and tyrosine[13]. Concentrations of these metabolites in the serum of patients with sepsis have been shown to be tens or hundreds of times higher than in healthy individuals, especially in the group of patients with a fatal outcome, so they are referred to as sepsis-associated aromatic microbial metabolites[24].
In inherited metabolic disorders associated with impaired amino acid metabolism, such as phenylketonuria, when the conversion of phenylalanine to tyrosine is disturbed, phenylalanine and its metabolites, PPA and PhLA (in anionic form), accumulate in the body in the blood and are excreted in the urine[25]. In turn, some disorders of tyrosine catabolism in the liver result in tyrosinemia due to the decreased activity of the enzyme p-hydroxyphenylpyruvate dioxygenase, which converts p-HPPA to HGA[26]. The accumulation of HGA in blood and urine is considered to be the most informative marker for the diagnosis of alkaptonuria, a rare autosomal recessive disorder of tyrosine metabolism associated with a deficiency in the activity of the enzyme homogentisate 1,2-dioxygenase[27, 28]. It has been found that urinary PhAA levels can be used as a biomarker of health status in an elderly population[29]. Increased levels of microbial metabolites of the aromatic amino acids PhAA, p-HPhAA, and other acids have been reported in patients with chronic renal failure and colorectal cancer[30]. Alterations in urinary aromatic amino acid metabolism have been reported as low-molecular-weight markers of prostate cancer[31]. The diagnostic utility of urinary PPA determination in alcoholic liver disease has been noted[32]. A correlation between the gut microbiota and the serum metabolite p-HPhLA, which in turn is associated with genetic predisposition to hepatic steatosis and fibrosis, has been reported[33]. A correlation has been found between an excess of several bacterial species in the gut microbiota and levels of circulating p-HPhLA, including bacterial species associated with advanced fibrosis[34]. Evidence is presented that p-HPhLA in the cerebrospinal fluid is a promising marker for predicting the development of secondary meningitis in the post-neurosurgical patients[35]. The role of PhAA in the cross-interference between the gut microbiota and obesity in women has been established[36]. There is evidence of a significant increase in serum and urinary levels of AMCAs in patients with celiac disease, ulcerative colitis and Crohn’s disease compared to healthy individuals[37]. Advanced urine test for organic acids (60 indicators, including p-HPhLA, p-HPPA, o-HPhAA, PhLA and HGA) by CMS is currently available in clinical laboratories. The main purpose of such analyses is to get an idea of the general state of human health and metabolic processes in the human body[38].
Therefore, numerous studies have repeatedly proved that qualitative and quantitative changes in the content of certain AMCAs in biological media of the human body are associated with the manifestation of certain diseases, so reliable quantitative determination of these compounds is of great interest for the purposes of the diagnostics and treatment of many human diseases and pathological conditions.
3. Methodological aspects of the determination of aromatic monocarboxylic acids in biological body fluids
In 1971, Linus Pauling et al.[39] pioneered the idea that all information about the functional state of an organism is reflected in the qualitative and quantitative composition of body fluids. To determine metabolically relevant organic acids in biological samples, various types of gas chromatography (GC), liquid chromatography (LC) and capillary electrophoresis (CE) are most commonly used, mainly in combination with mass spectrometry (MS) or tandem mass spectrometry (MS/MS), as well as chemiluminescence, fluorimetry and UV detection[40-46]. The analysis of biological matrices by the above mentioned methods requires prior sample preparation, which may include protein removal, isolation/concentration of analytes followed by derivatization (Figure 2).
3.1. Gas chromatography-mass spectrometry methods
Gas chromatography-mass spectrometry (GC-MS) is the most common method for the determination of small molecules, especially AMCAs. Therefore, the number of methodological studies describing the separation and determination of various AMCAs in biological fluids by GC-MS is quite large[8-11]. AMCAs have volatility and thermostability that allow their determination by GC without prior chemical modification when polar capillary columns are used. However, the presence of polar hydroxyl and/or carboxyl functionalities in AMCAs results in asymmetric chromatographic peaks when analyzed using common non-polar capillary columns. In addition, the AMCA mass spectra comprise low non-selective characteristic m/z values and this reduces the sensitivity of the analysis when using a mass spectrometric detector. These features of the analysis of the unmodified AMCAs have led to their chemical modification in the sample preparation to obtain, e.g., silyl or methyl derivatives (derivatization reaction), becoming the most common step prior to a GC-MS analysis. The resulting derivatives (ethers) show much lower polarity, which improves their chromatographic performance, and have higher molecular weight, which leads to a shift of their characteristic m/z values into the higher and mass-selective range. The choice of a derivative type affects the sample preparation: in the case of silyl derivatives it is important to remove residual water from the sample after extraction of the target components from the matrix; methylation, on the other hand, can be carried out in the presence of residual water or directly in the aqueous sample[47].
Silylation, which is the nucleophilic substitution of an active hydrogen atom with an alkylsilyl group by the SN2 mechanism, is most often used for the AMCA derivatization due to its simplicity, relative rate and versatility. The most common silylation reagents are N,O-bis(trimethylsilyl)trifluoroacetamide (BSTFA) or its mixture with trimethylchlorosilane, and N-methyl(trimethylsilyl)trifluoroacetamide (MSTFA) to form trimethylsilyl derivatives, and N-methyl-N-(tert-butyldimethyl-silyl)trifluoroacetamide (MTBSTFA) to form tert-butyl-dimethylsilyl derivatives. The trimethylsilyl derivatives hold leading positions due to their lower boiling points than tert-butyldimethylsilyl derivatives, resulting in earlier elution of analytes from the chromatographic column and shorter analysis times. Derivatization of AMCAs can be carried out either after evaporating the sample to dryness or directly in the organic extract[48]. In addition, derivatization can be performed either as a separate sample preparation step (at room temperature or in a thermostat followed by sample cooling) or directly in the injection system of a gas chromatograph with temperature programming capability[49].
Sample preparation is an important step in the extraction of AMCAs from biological samples for the subsequent GC-MS analysis. Pre-precipitation of matrix proteins is often performed using various organic (methanol, trichloroacetic acid) or inorganic (inorganic acids, salts) precipitators. When selecting sample preparation conditions, it is important to consider the need for conversion of analytes to the organic phase and concentration. The main types of sample preparation used in the case of AMCAs are liquid-liquid extraction and solid-phase extraction.
The use of liquid-liquid extraction can be characterized as follows:
— a wide range of organic extractants allows the selection of the most suitable extractant for analyte extraction depending on the application (diethyl ether, ethyl acetate, methyl tert-butyl ether, etc.);
— improving the characteristics of the extraction of aromatic acids into the organic phase is achieved by acidifying and/or salifying the initial aqueous sample, which makes it possible to convert the acids from anionic to molecular form and/or increase the ionic strength of the initial solution;
— sample preparation does not require expensive equipment and expendable materials; the main sample preparation equipment is a shaker (Vortex or similar), centrifuge, sample drying system;
— automating the process is extremely difficult and requires significant operator involvement at all stages;
— reducing sample preparation time is problematic because of the most common double extraction, i.e. two cycles of ‘mixing – centrifugation – sampling of organic phase’.
Methods using liquid-liquid extraction of p-HPhLA, p-HPPA, o-HPhAA, PhAA, PhLA, and HGA with diethyl ether, methyl tert-butyl ether, and others are described[50, 51]. The modified technique of liquid-liquid extraction with ethyl acetate followed by derivatization with BSTFA is actively used in clinical laboratories for GC-MS determination of 28 organic acids in urine, including p-HPhLA, p-HPPA, o-HPhAA, PhLA, and HGA[38].
An alternative method of sample preparation is solid phase extraction, which can be implemented in its classical mode using sorption cartridges or in more modern modifications using solid-phase microextraction (SPME) or microextraction by packed sorbent (MEPS)[52, 53]. The MEPS technique can be implemented as a semi-automatic programmable pipette or as a fully automated device integrated into a GC-MS system[54, 55]. The characteristics of solid-phase extraction are as follows:
— a wide range of sorbents (activated carbons, silica gel modified with alkyl[49, 51, 54], phenyl, nitrile groups; polymers; hypercross-linked polymers[56], etc.) allows the selective extraction of analytes depending on their chemical and physical properties;
— it is possible to concentrate the sample using modern variants of SPME or MEPS, while reducing the volume of extractants;
— the application of solid-phase extraction systems (including under reduced pressure) using sorbent cartridges (usually disposable) or specialized equipment and expendable materials for SPME or MEPS.
In the case of AMCAs, the difference in their polarity should be taken into account. For example, PhPA and PhAA have a lower polarity than PhLA and other hydroxy acids such as p-HPhAA and p-HPhLA, which is particularly noticeable in their group extraction or sorption.
As with any analytical method, GC-MS requires preliminary analysis of pure chemical standards of the analytes to determine retention time (index) and study of the mass spectrum to find the most characteristic m/z values. Next, the most appropriate internal surrogate standards for quantitative analysis are selected and added to the sample in the first steps, for the following reasons:
— the most preferred type of internal surrogate standard for CMS methods is an isotopic analogue of the original analyte (deuterated or 13C isotope-labelled); such a standard will almost completely compensate for analyte loss during the preliminary steps of sample preparation, as it has identical chemical properties to the analyte and will have different mass spectra despite overlapping chromatographic peaks;
— in the absence of isotopic analogues of the analytes, the choice of an internal surrogate standard is based on its absence in the initial matrix, retention time (index) different from that of the target analytes and m/z values different from the background characteristic values in the mass spectrum[57].
The different polarity of AMCAs also requires the use of different internal surrogate standards for their quantification. The use of several different standards has been reported: D5-benzoic acid for the determination of the more non-polar analytes (PhAA, PhPA) and D3-p-HPhLA or 3,4-dihydroxy-benzoic acid for the more polar representatives (PhLA, p-HPhAA, p-HPhLA, etc.)[48].
In addition to surrogate standards that allow the control of the analyte extraction and derivatization, it is common in GC-MS to use an internal standard that is added to the sample immediately prior to GC-MS analysis, thus controlling the analysis per se. The choice of such a standard is also determined by its absence in the original matrix and its high sensitivity in mass spectrometric detection. Such a standard may be, e.g, hexachlorobenzene[57].
Special attention should be paid to the determination of the optical forms of AMCAs with chiral centers, such as PhLA and p-HPhLA. The enantiomeric ratio of chiral metabolites is an important parameter to gain insight into metabolic processes. In the implementation of enantioselective GC-MS analysis, a column coated with a suitable chiral stationary phase is used to separate the optical isomers of PhLA and p-HPhLA in a single run[58].
All above-described sample preparation methods allow the detection of AMCAs in urine, serum or plasma, cerebrospinal fluid in a sample volume of less than 500 mL (in the case of modern sample preparation methods such as MEPS even in 50 mL of sample) at concentrations characteristic of both healthy donors (0.1 – 2.0 mmol L–1) and patients with various pathologies (more than 2.0 mmol L–1). The results of the individual studies specifying the particular features of sample preparation, method of analysis, type of matrix and analytical characteristics of the techniques developed, are presented in Table 1.
The reliability of GC-MS data is beyond doubt since retention time/retention time/index and mass spectral data are used for identification and can be compared with mass spectral libraries. At the same time, the main factors limiting the active implementation of the developed procedures in the clinical use are the high cost of the equipment, the need to employ a skilled specialist for sample preparation and data analysis, and, despite the possibility of automating sample preparation, the duration of the determination, which includes the time for sample preparation (at least 30 minutes, and on average 60 – 90 minutes) and GC-MS analysis (15 – 30 minutes).
3.2. Liquid chromatography methods
The use of high-performance liquid chromatography coupled to mass spectrometry (HPLC-MS) allows the determination of metabolites at very low concentrations in samples. However, the sensitivity of the analysis depends on many factors, including the type of mass analyzer used. In the last decade, laboratories involved in testing for metabolic disorders and metabolic analysis have been using tandem mass spectrometry, which has the advantage of increased sensitivity and specificity when using multiple reaction monitoring techniques. For metabolic profiling, including the search for unknown compounds, high-resolution time-of-flight or quadrupole time-of-flight mass spectrometers are used to determine accurate mass and isotope distributions, and low-resolution triple quadrupole mass analyzers are used in routine analysis or in the development of validated procedures for the detection of one or more metabolites[57].
The HPLC-MS/MS method with electrospray ionization at atmospheric pressure is one of the most important analytical methods in practical medicine worldwide. However, a peculiarity of the analysis of biological matrices by mass spectrometry using an ionization source at atmospheric pressure is the presence of the matrix effect, which can affect the ionization, suppressing or enhancing it, and thus distorting the information about the true concentration of the analyte. This effect should be considered at the stage of developing the method for analyte quantification and, if it occurs, alternative matrices should be sought or simpler solvents should be explored as matrices[59]. It is also important to ensure that the matrix effect does not influence the internal standards used in method development.
In addition to the matrix effect, the choice of an alternative matrix may be related to the need to consider the endogenous content of AMCAs in biological samples when developing the procedure[60]. To determine the content of an unknown compound in samples, it is necessary to construct a calibration curve using a matrix similar to that in the samples. However, in the case of endogenous compounds, this can be difficult because the detection and quantitation limits cannot be lower than the lowest concentration of the compound detected, e.g., in samples from healthy donors. In addition, the serum AMCAs can vary widely depending on individual characteristics and health status, so it is important to develop techniques that allow quantification of these compounds at different levels, lower or higher than in healthy individuals. This may require an AMCA-free matrix; in some cases, conventional solvents can be used[61, 62].
For example, in a recent study a sensitive method for the determination of aromatic amino acid metabolites, including PhPA, PhLA, p-HPhAA, and p-HPhLA, in serum by precipitation of serum proteins with methanol and analysis by HPLC-MS/MS was developed and validated. Recovery of all analytes reached 100% and no matrix effects were observed with protein precipitation, allowing the use of deionized water as matrix to construct a calibration curve[63]. Previous studies have described the quantitative analysis of 20 microbial metabolites, including a number of AMCAs, in rat serum, urine and faeces[61] using methanol as a matrix for constructing a calibration curve, and the analysis of more than 50 different AMCAs, including their isomers, in human serum, urine and cerebrospinal fluid by HPLC-MS/MS 8 using artificial cerebrospinal fluid for validation and calibration.
The presence of carboxyl and/or one or more hydroxyl groups in AMCAs allows their determination by reversed-phase HPLC without prior derivatization. Sample preparation, including protein precipitation, extraction, concentration or pH modification, remains an important preliminary step. Simple and rapid precipitation of proteins with organic precipitants followed by centrifugation and analysis of the supernatant can be used for HPLC-MS or HPLC-MS/MS, significantly reducing sample preparation time[64]. However, as with GC-MS analysis, inferior sample preparation methods using liquid-liquid extraction or solid phase extraction have been described[65]. Their use may be justified when using other detection methods, such as a universal UV detector, which may give false positives if there is insufficient separation of chromatographic peaks and/or a significant influence of interfering matrix components. The results of some studies, including the type of matrix, sample preparation method, features of HPLC analysis and analytical characteristics of the developed methods, are presented in Table 2.
As with GC-MS analysis, the enantiomeric resolution of analytes with chiral centers is also possible in HPLC using specialized columns, which have been used in particular for the resolution of L, D enantiomers of PhLAs[66].
It is important to demonstrate the reliability and suitability of the methods developed in order to obtain reproducible and accurate results, so method validation is essential. The US Food and Drug Administration (FDA)[67] and the European Medicines Agency (EMA)[68] have previously published guidelines describing general principles and recommended approaches for the validation of bioanalytical methods. In January 2023, the International Council for Harmonization (ICH) issued new guidance on the validation of bioanalytical methods, including CMS, which unifies and clarifies previously issued recommendations. The guidance includes the definition of several analytical parameters that must be demonstrated to meet minimum criteria before a method can be considered analytically validated. The method should demonstrate selectivity and specificity with which the analytes of interest can be measured without the influence of extraneous compounds or matrix effects; it should allow accurate and precise measurement of the nominal analyte concentration without changes due to sample carry-over, degradation or dilution effects. It also provides guidelines for the validation of endogenous analytes. Despite many studies reporting the determination of AMCAs in plasma and serum, few of the methods used have been properly validated[62, 63, 69, 70].
The introduction of sensitive and specific HPLC-MS/MS methods into clinical practice appears to be more feasible than GC-MS because of the availability of the necessary equipment in multidisciplinary medical centers of regional importance, especially for amino acid screening. However, the number of such centers is limited and the potential diagnostic value of AMCAs includes their monitoring in patients in the intensive care units. In such a case, equipping clinical laboratories with appropriate equipment is problematic due to its high cost and the need for a skilled specialist, similar to the use of GC-MS.
3.3. Capillary electromigration methods
Nowadays, capillary electromigration methods — capillary zone electrophoresis and electrocapillary chromatography (EC) are of growing importance in the analysis of biological fluids. The use of EC for the determination of short-chain aromatic carboxylic acids in body fluids is characterized by rapidity, ultra-small sample volumes and low solvent consumption[71-74]. Remarkably, in ideal separation in CE systems, the zone broadening of analyte peaks is explained only by molecular diffusion, in contrast to HPLC separation where zone broadening, even in the ideal case, is determined by several factors (longitudinal diffusion, mass transfer rate, etc.). To achieve high sensitivity of the analysis, either on-line (continuous) sample concentration or high sensitivity detectors are usually used. Thus, CE-MS and CE-MS/MS systems represent a promising platform for the diagnosis of metabolic disorders, since they allow the qualitative and quantitative determination of a wide range of polar metabolites in biological fluids without laborious sample processing[75, 76]. The analytical characteristics of a number of CE techniques used for the determination of individual AMCAs in urine are summarized in Table 3.
Much attention has been given in the scientific literature to the profiling of clinically relevant metabolites in human urine by capillary electrophoresis with amperometric detection (CE-AD)[82-84]. In particular, the developed CE system with on-line amperometric detection has been proposed for the separation and determination of tyrosine and its metabolites, including p-HPPA and HGA[82]. A graphite disc electrode was used as the working electrode as part of a wall-jet flow cell. CE and MEKC (Micellar Electrokinetic Chromatography) methods in combination with non-contact conductivity determination with capacitive coupling (C4D) and amperometric detection were proposed[83, 85].
In general, CE is recognized as a powerful analytical separation method that allows the detection of important changes in the metabolic profile of body fluids relatively quickly and efficiently. The main advantages of the method relevant to this problem are the ability to separate low-molecular-weight organic molecules in complex matrices without sample pre-treatment and the small sample volume (1 – 100 nL), which is of particular interest for body fluids such as tears, cerebrospinal fluid and even newborn blood. The main advantages of CE also include high resolution, speed and lower cost of analysis due to the use of very inexpensive capillaries (as opposed to expensive HPLC capillaries). There is growing interest in the use of EC, which combines the advantages of capillary zone electrophoresis and HPLC, for biomarker screening[86]. Of interest is the development of the dual detection concept, in particular the use of a CE system with amperometric and mass spectrometric detection[87]. An important advance in the development of CE seems to be the possibility of detection using microchips[88, 89].
4. Portable detection tools for aromatic monocarboxylic acids and potential ways for their development
Today we rely heavily on technology and increasingly use it to monitor our own health. Despite the undeniable advantages of combining chromatographic and electrocapillary separation with MS or MS/MS, the major drawbacks of the proposed methods are the complexity of the analytical system, the need for expensive equipment and highly skilled personnel, making them of little use for routine use at points of care. It is also important to note that in a number of cases it is more appropriate to use selective techniques and sensor devices that allow the isolation and determination of a specific biomarker in different biological fluids. This accounts for the interest in the development and introduction into practice of portable (handheld) devices and screening tests that can be used to perform rapid real-time diagnostic tests with a high degree of reliability.
4.1. Diagnostic screening tests
Several standardized screening tests have been developed to detect inherited or acquired disorders of phenylalanine and tyrosine metabolism in body fluids[90-92]. For example, the laboratory diagnosis of phenylketonuria in children includes the detection of elevated phenylalanine in the blood and the presence of PPA in the urine (Fehling’s test). The analysis is based on the ability of the enolic form of PPA, which accumulates in the blood and is excreted in large amounts in the urine, to form a complex with trivalent iron ions, which is coloured blue-green[93]. Typically, the amount of PPA in the daily urine of a phenylketonuria patient is 0.05 – 3.0 g or 0.3 – 18 mmol L–1. There are a number of commercially available sets of indicator test strips ‘Fenistix’, ‘Biophan’, etc. for the determination of urinary PPA.
Another extraction-photometric test for the detection of urinary PPA is based on a colour reaction with 2,4-dinitrophenylhydrazine in alkaline medium to form yellow-orange pyruvic acid 2,4-dinitrophenylhydrazone[94].
Screening tests for the detection of HGA in the urine of alkaptonuria patients are based on its oxidation by atmospheric oxygen in an alkaline environment to form a blue-violet coloured pigment product or its ability to reduce ammonium molybdate to form molybdenum blue[95].
4.2. Electrochemical sensors and biosensors
The use of electrochemical sensors and biosensors appears to be a promising trend for the sensitive detection of low-molecular-weight biomarkers, including AMCAs. Electrochemical sensors have a number of advantages such as easy pre-processing, short analysis time, low cost and portability. However, the electrochemical determination of AMCAs in body fluids is still rarely reported in the scientific literature. The electrochemical behaviour of PhAA (in the form of phenyl acetate) was studied in the potential scanning range from –1.0 to +1.0 V in 0.1 M KCl solution[96, 97]. Based on these findings, an amperometric sensor consisting of a glassy carbon electrode modified with CuGeO3 nanowires was fabricated. The peak current height varies linearly with increasing acid concentration from 0.01 to 2 mmol L–1 and the detection limit is 9.1 mmol L–1. The sensor has good reproducibility, stability and sensitivity.
The determination of PPA in urine is of great diagnostic significance in alcoholic liver disease. A phenylpyruvate-selective membrane electrode has been first developed which allows the in situ determination of phenyl pyruvate in urine[98]. The sensor is based on a plasticized polyvinyl chloride membrane containing heptyl 4-trifluoroacetylbenzoate (ETH 6010) and methyltridodecylammonium chloride. A 1.0 mM solution of sodium phenyl pyruvate in 0.01 mM NaCl was used as the internal blank solution. The range of detectable phenyl pyruvate concentrations is 2 – 12 mmol L–1. The sample volume is 200 mL. It should be noted, however, that liquid-contacting membrane ion-selective electrodes (electrodes with an internal reference solution) have a number of disadvantages, such as short lifetime, drying of the internal solution, potential instability and the need for frequent calibration of the device, which significantly limit their practical application. It is therefore not surprising that sensors based on polymer membranes with a solid contact (instead of an internal blank solution) are a promising trend in the field of modern potentiometry[96].
It is clear from the literature review that there is a growing global interest in the development of highly selective methods for the determination of target molecules[97-99], including those of aromatic structure, using MIPs, which allow the formation of specific cavities for analyte binding and recognition in the biomatrix[100, 101]. MIPs are a class of synthetic polymeric macromolecules that possess stereospecific target recognition capabilities and are now being considered as biomimetic recognition agents[102]. Although the mechanisms of imprinting are not fully understood, MIPs have already established themselves as artificial receptor materials in electrochemical sensors for the detection of small molecules that are biomarkers of various diseases[103-105]. Recognition mechanisms between imprinted polymers and various biomarkers such as signalling molecules, microbial toxins, viruses, bacterial and fungal cells have been considered. However, to the best of our knowledge, there are still no works on the application of MIP as an electrode active material for the selective determination of the AMCAs considered in this review.
The development of biosensors is also a promising solution to this problem[91, 92]. For example, tri-colour whole-cell biosensors have been developed for the simultaneous detection of three analytes (phenylalanine, PhAA and phenylethylamine) in phenylketonuria patients[106]. The designed whole-cell sensors were successful in analyzing urine samples without pre-treatment. The combination of three sensor strains together allowed semi-quantitative assessment of three analytes by unique colour appearances without cross-interference. Despite its simple design, the proposed device shows specificity and sensitivity. The proposed whole-cell sensors were successful in analyzing urine samples without pre-treatment. The combination of the three sensor strains together allowed semi-quantitative assessment of the three analytes by unique colour appearances without cross-interference. The sensors have been incorporated into a microchannel chip to minimize reagent consumption and provide portability. A whole-cell biosensor has recently been developed that shows an independent response to exogenous PAA with a limit of detection of 9.54 nmol L–1, the highest level of sensitivity reported to date[107]. In addition, the biosensor has a high specificity for PAA compared to the natural hydroxylated forms of this compound. Obviously, molecular design and nanotechnology in the creation of new (bio)sensors should be considered as one of the priority ways to create microanalytical systems for the purposes of everyday medical diagnostics[108].
5. Conclusion
The quantification of aromatic molecular markers in biological fluids is of great interest for the diagnosis, monitoring and prognosis in the treatment of a number of severe diseases. It is important to note the modern achievements of GC-MS and HPLC-MS methods, which have made it possible to study the profile of aromatic metabolites in human blood, to identify aromatic amino acid metabolites characteristic of various diseases and pathological conditions, and to establish diagnostically significant concentrations of the desired metabolites with high accuracy and reproducibility. The results of basic research, obtained using the most advanced chromatography-mass spectrometry equipment, comparing biological samples from patients with different diseases and healthy donors, provide a reliable basis for future prospective and applied research. A review of the literature shows that there is a growing need for the development of new technologies that can measure the concentration of specific diagnostically relevant aromatic biomarkers in real time. In the field of rapid diagnostics, various scientific groups are looking for optimal solutions that would increase availability and ensure widespread clinical implementation by reducing the labor and test costs. Among the promising development trends in the methodology of AMCA determination, the development of MIP-based optical and electrochemical sensors deserves special attention, since it has great potential for widespread practical use in medicine.
6. Abbreviation
AMCA — aromatic monocarboxylic acids;
BSTFA — (N,O-bis-trimethylsilyl)trifluoroacetamide;
CE — capillary zone electrophoresis;
CMS — chromatography-mass spectrometry;
EC — electrocapillary chromatography;
GC — gas chromatography;
GC-MS — gas chromatography-mass spectrometry;
HGA — homogentisic acid;
HPLC-MS — high-performance liquid chromatography coupled with mass spectrometry;
p-HPhAA — 2-(4-hydroxyphenyl)acetic acid;
p-HPhLA — D,L-3-(4-hydroxyphenyl)lactic acid;
p-HPPA — 3-(4-hydroxyphenyl)pyruvic acid;
LC — liquid chromatography;
MEPS — microextraction by packed sorbent;
MIP — molecularly imprinted polymer;
MS — mass spectrometry;
MS/MS — tandem mass spectrometry;
MSTFA — N-methyl(trimethylsilyl)trifluoroacetamide;
MTBSTFA — N-methyl-N-(tert-butyldimethylsilyl)-trifluoroacemide;
PhAA — 2-phenylacetic acid;
PhLA — D,L-3-phenyllactic acid;
PhPA — 3-phenylpropionic acid;
PPA — 3-phenylpyruvic acid;
SPME — solid-phase microextraction.
References
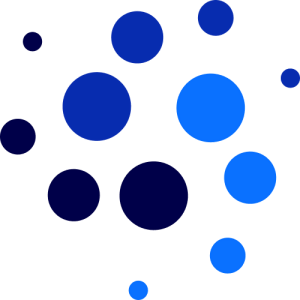
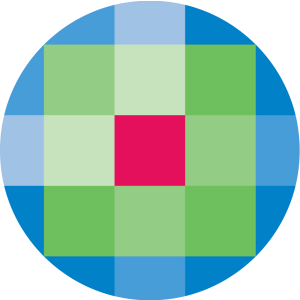
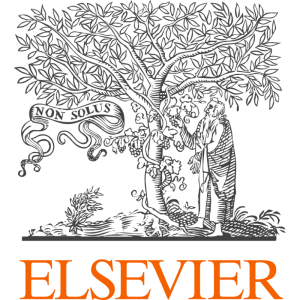
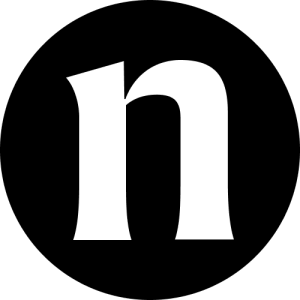
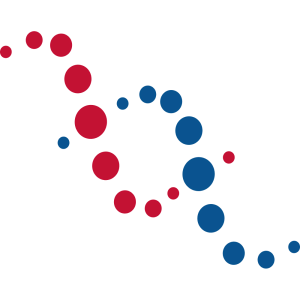
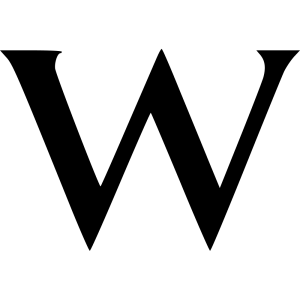
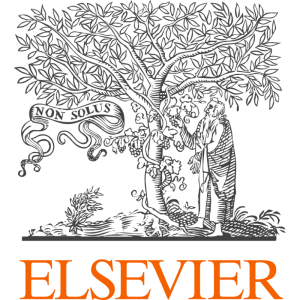
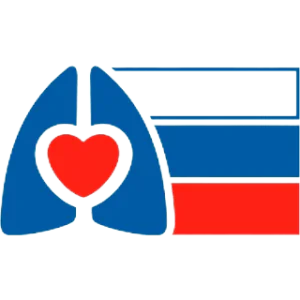
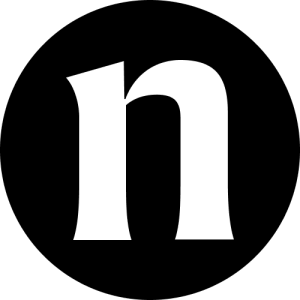
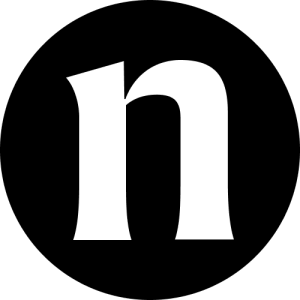
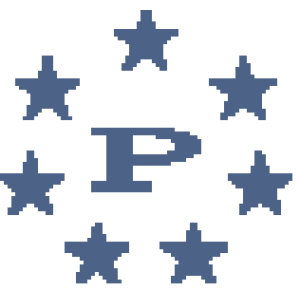
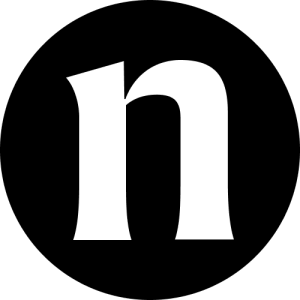
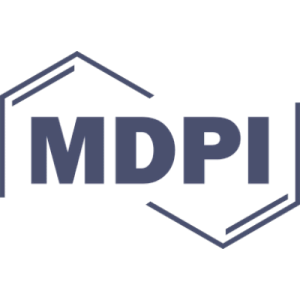
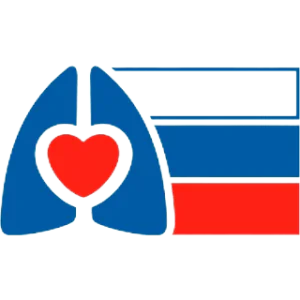
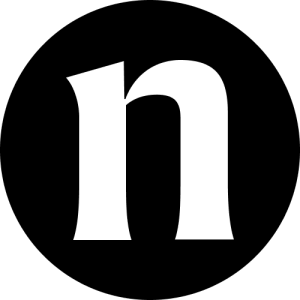
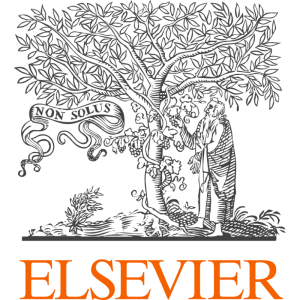
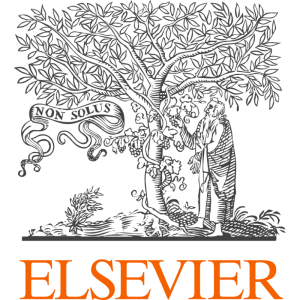
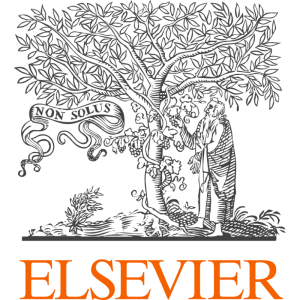
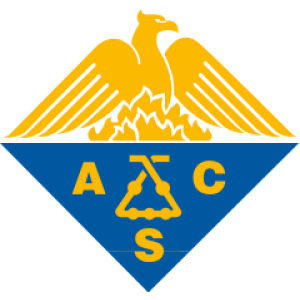
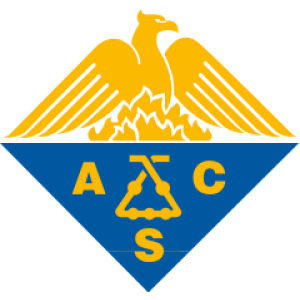
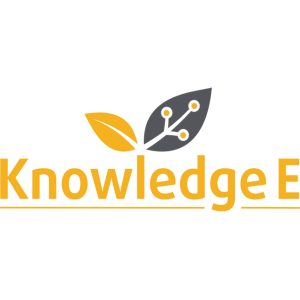
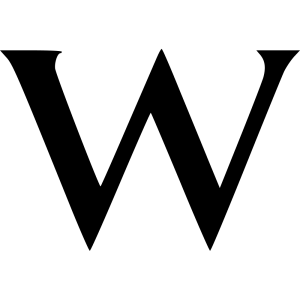
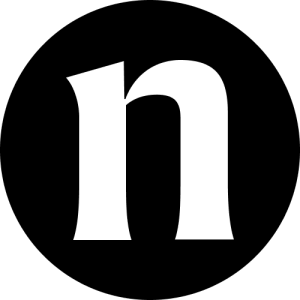
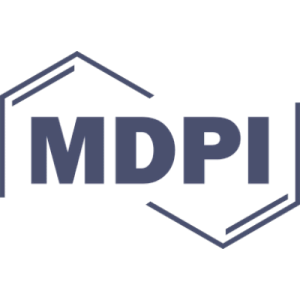
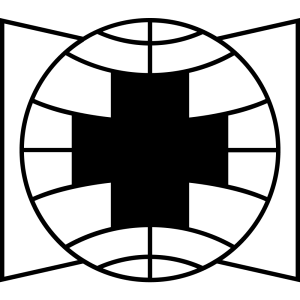
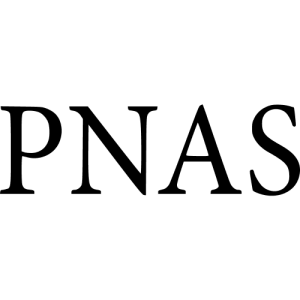
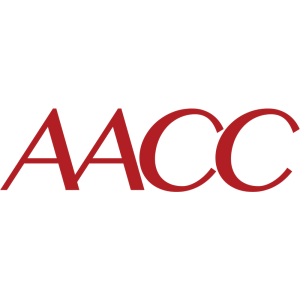
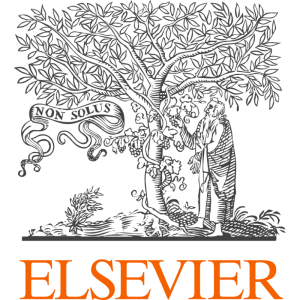
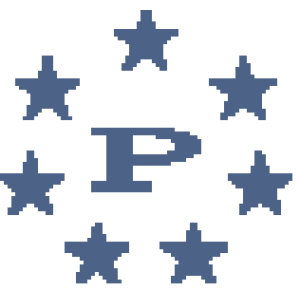
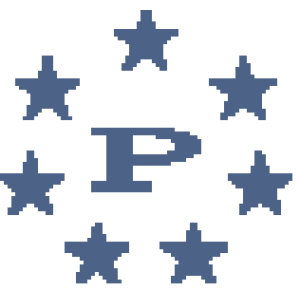
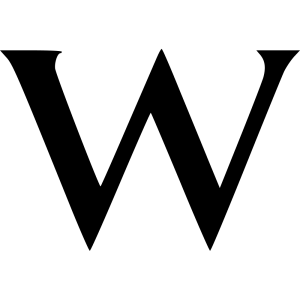
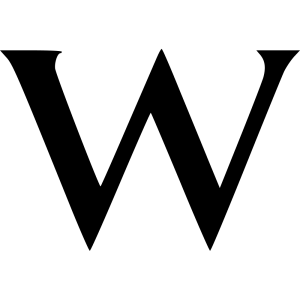
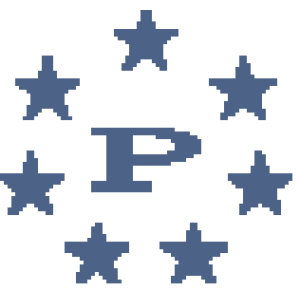
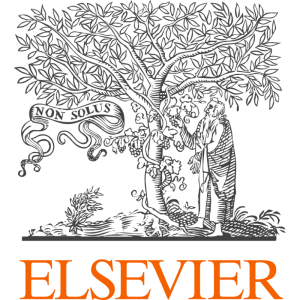
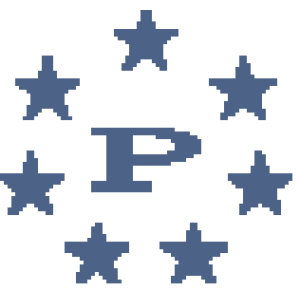
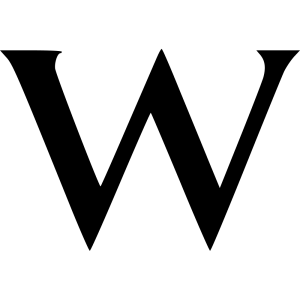
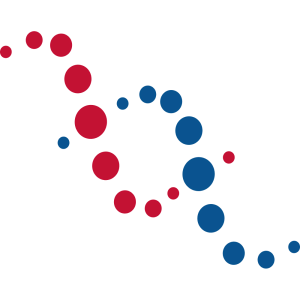
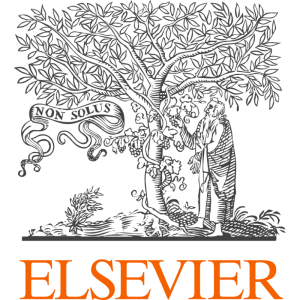
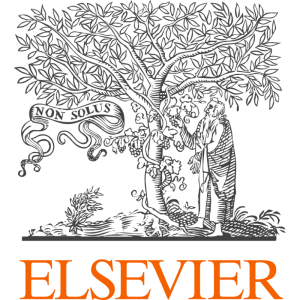
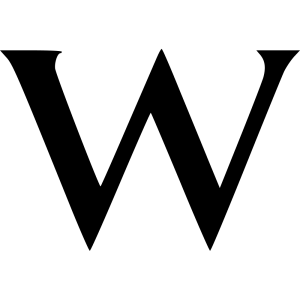
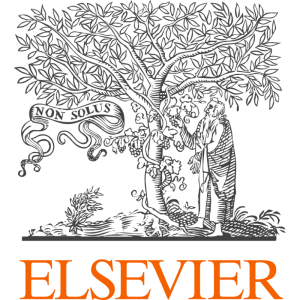
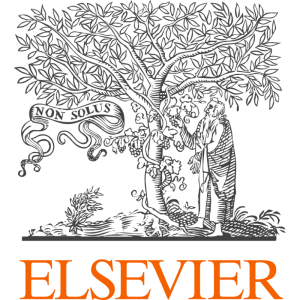
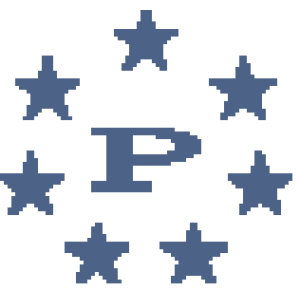
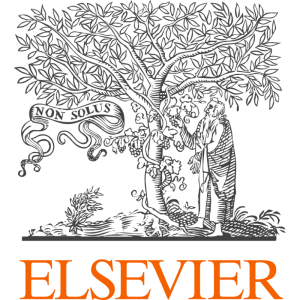
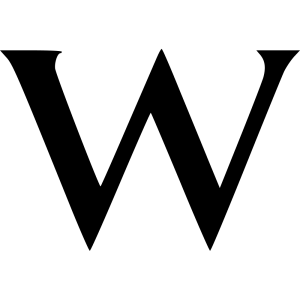
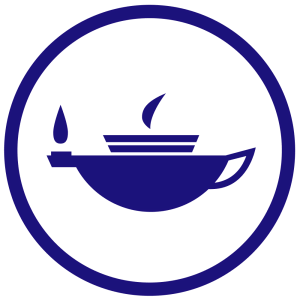
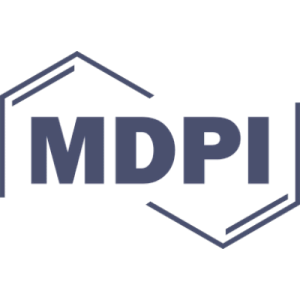
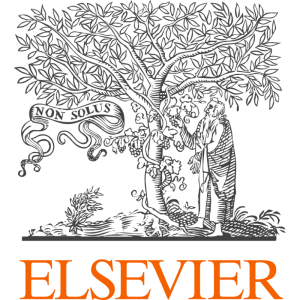
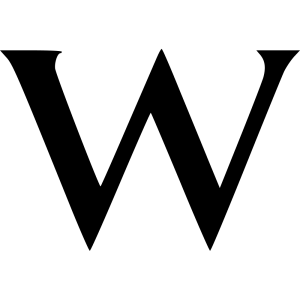
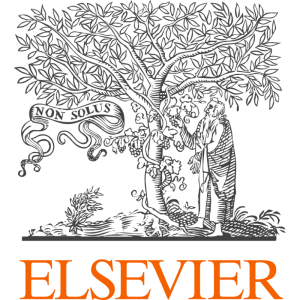
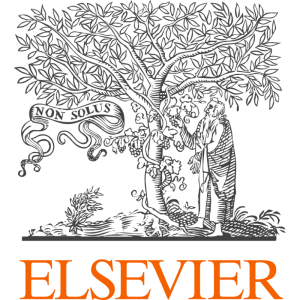
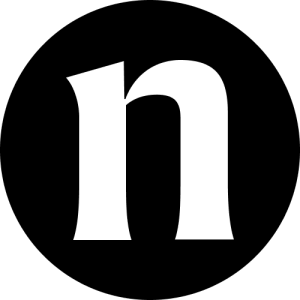
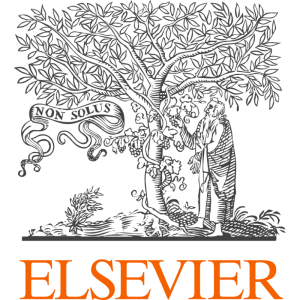
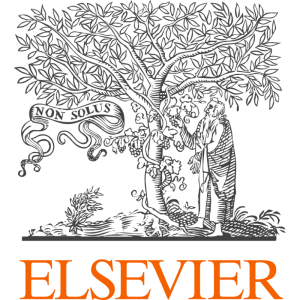
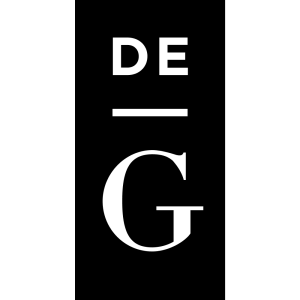
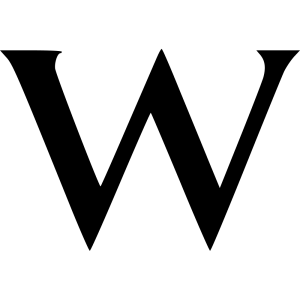
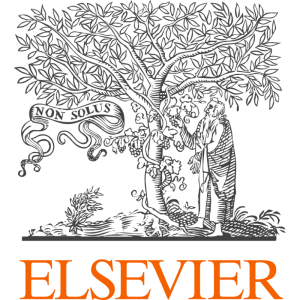
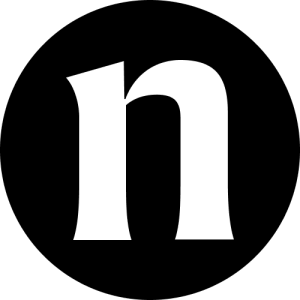
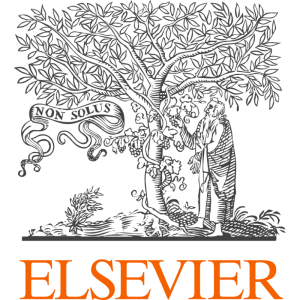
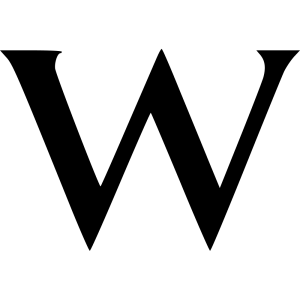
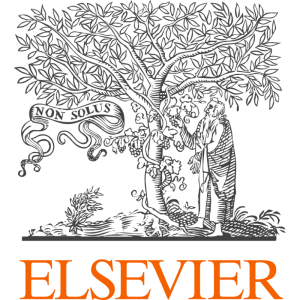
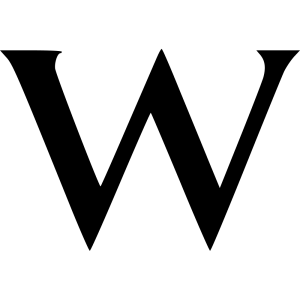
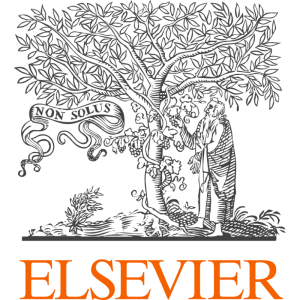
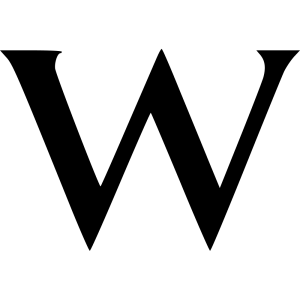
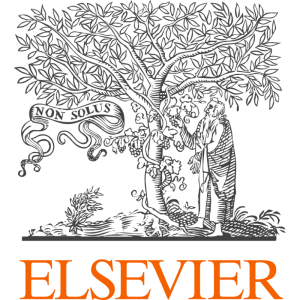
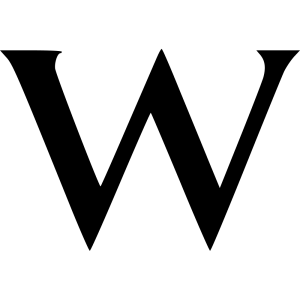
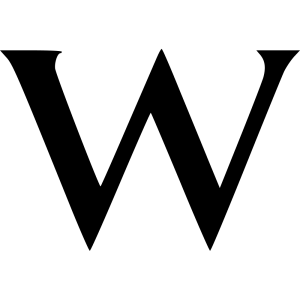
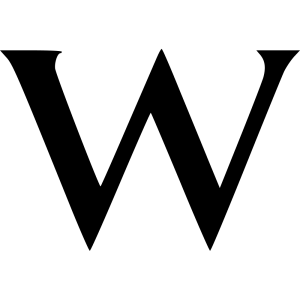
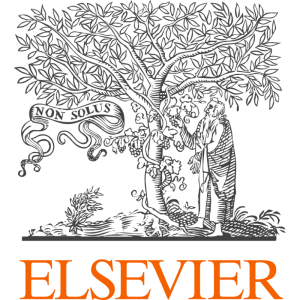
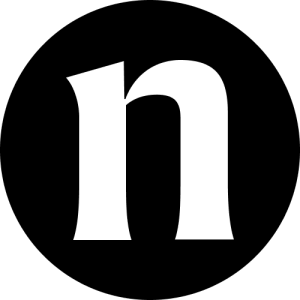
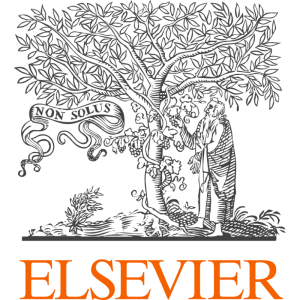
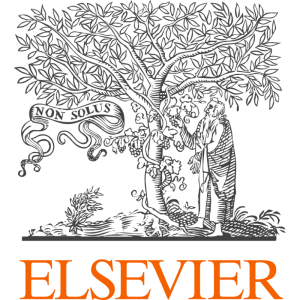
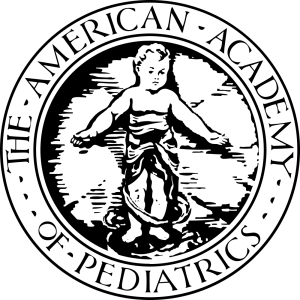
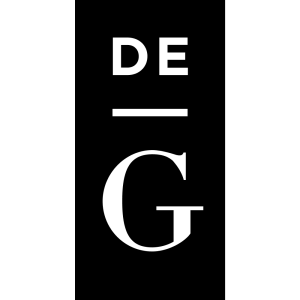
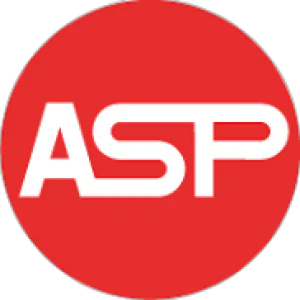
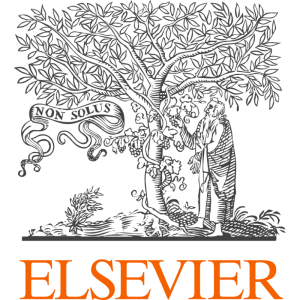
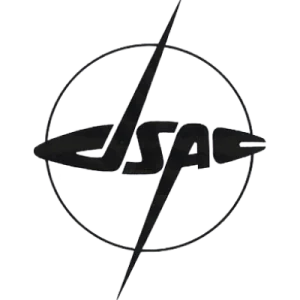
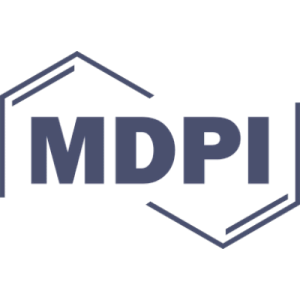
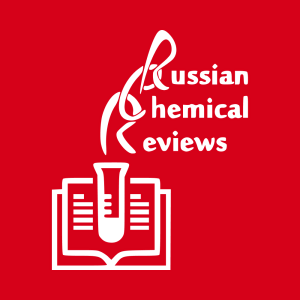
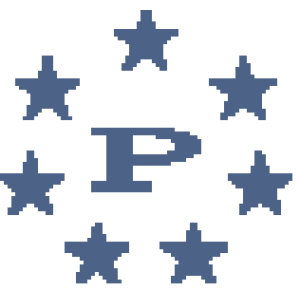
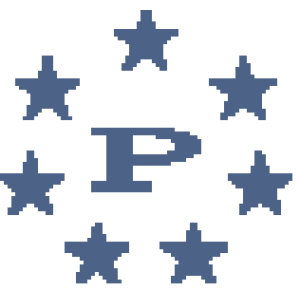
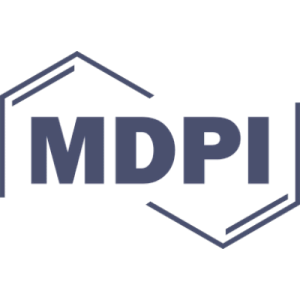
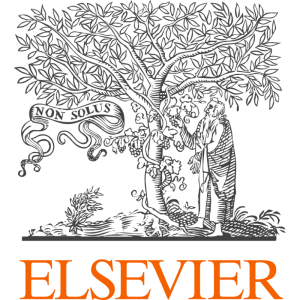
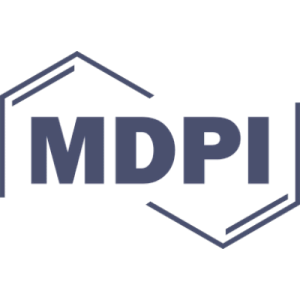
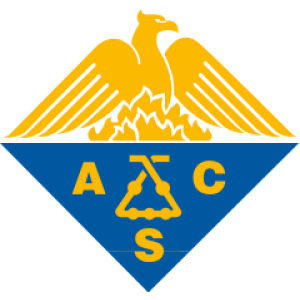
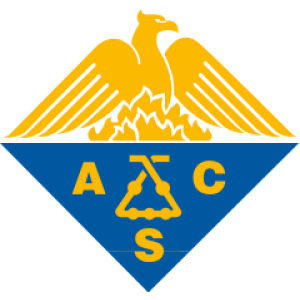
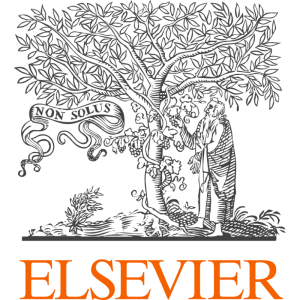