Keywords
Abstract
The interest of the global scientific community in the problems of CO2 utilization and returning to the carbon cycle has markedly increased in recent years. Among various CO2 transformation processes, photocatalytic reduction is one of the most promising. Currently, much attention is paid to photocatalysts based on graphitic carbon nitride, since the use of g-C3N4 makes it possible to perform CO2 reduction under visible or solar light irradiation. To increase the reduction efficiency, g-C3N4 is subjected to various modifications with the most popular and promising approach being the synthesis of composite photocatalysts based on g-C3N4 with other semiconductors to form heterostructures. Depending on the type of semiconductor, transfer of photogenerated charge carriers in these systems can occur by various mechanisms, which largely determine the course of the process and the rates of formation of reaction products. This review addresses studies on the synthesis of composite photocatalysts based on g-C3N4, with emphasis being placed on the mechanisms of charge carrier transfer and the distribution of products of CO2 reduction.
The bibliography includes 235 references.
1. Introduction
A primary task of rational management and protection of the environment is to reduce the concentration and emissions of greenhouse gases. The development of methods for reducing concentrations of greenhouse gases is the subject of many studies[1-3], with much attention being paid to utilization of carbon dioxide, the major component of greenhouse gases[4-8]. Today, there are several industrial processes for converting СО2 to valuable products such as urea, salicylic acid, ethylene carbonate and methanol. Due to the high thermodynamic stability of the CO2 molecule, traditional processes of CO2 conversion to products are carried out at high temperature and high pressure, e.g., synthesis of urea from СО2 and NH3 takes plates at a temperature of 185 °С and a pressure of 150 bar[9-11], which substantially increases the energy expenditure and decreases the economic attractiveness of these processes, despite the readily available feedstock.
For this reason, of particular interest are the ways to decrease the energy consumption of CO2 conversion processes. One of the approaches to address this task is the photocatalytic reduction of CO2, which can occur under ambient conditions[7, 12]. This process is based on the use of renewable resources, solar light and water, which makes photocatalytic reduction a promising method for CO2 utilization, but its industrial implementation is primarily limited by the lack of efficient photocatalysts. Nevertheless, photocatalytic reduction of CO2 complies with the principles of sustainable development and, in the future, it may become the most facile and inexpensive way for decreasing the CO2 concentration in the atmosphere[12, 13]. Moreover, this process gives organic compounds such as CH4, CH3OH and HCOOH [equations (1),(2),(3),(4), (5)], standard electrode potentials vs. NHE at pH = 7 are given], which can be used as synthetic fuel and in chemical industy[12, 14-17].
In recent years, the attention of researchers has been attracted to a new polymer semiconductor, graphitic carbon nitride g-C3N4 (Figure 1), materials based on which can be used in various photocatalytic reactions[18, 19]. It is found that g-C3N4 has a planar structure based on heptazine or triazine units[20], in which carbon and nitrogen atoms are sp2-hybridized. This promising semiconductor has high chemical and thermal stability; in addition, g-C3N4 can be obtained from readily available precursors by simple methods[21, 22]. As compared with many semiconductors traditionally used in photocatalysis, for example TiO2, g-C3N4 has a rather narrow band gap (2.7 eV); in combination with the highly negative position of the conduction band (–1.3 V vs. NHE), this results in light absorption over a broad range of wavelengths and a high reduction potential of photogenerated electrons[23, 24]. In addition, g-C3N4 has a number of properties inherent in two-dimensional materials such as high mobility of charge carriers, high surface area to volume ratio and the presence of quantum size effect[25, 26]. The drawbacks of g-C3N4 include fast recombination of photoinduced electron – hole pairs[27] and low adsorption ability, which hampers any heterogeneous catalytic process[28, 29]. A popular and efficient approach to overcome these difficulties is to form heterostructures with other semiconductors or materials, which would enhance the efficiency of charge carrier separation and the adsorption properties.
It is known that photocatalysts are activated upon absorption of light quanta with energy equal to or exceeding the size of the band gap energy of the semiconductor. As this takes place, the electron migrates from the valence band to the conduction band, and an electron vacancy (hole) appears in the conduction band. Then the photogenerated electrons and holes can either migrate to the photocatalyst surface and be involved in redox reactions with adsorbed reagents or recombine in the photocatalyst bulk to release heat. The recombination of electron – hole pairs sharply decreases the efficiency of the photocatalytic reaction; therefore, an important task of photocatalysis is separation of the photogenerated charge carriers to increase their lifetime.
On contact of two semiconductors, the difference between the Fermi levels induces the electron transfer, giving rise to a built-in electric field, which, in turn, promotes the transfer of photoinduced charge carriers[30-32]. The charge carrier separation in composite structures can proceed by different mechanisms. The classical schemes of charge carrier transfer between two semiconductors include type I and II heterojunctions. Type I heterojunction appears when the conduction band (CB) of one semiconductor (SC 2 in Figure 2а) is located lower, while its valence band (VB) is located higher in energy than these bands of another semiconductor (SC 1 in Figure 2а). In such a structure, electrons and holes would migrate from the wide-band-gap semiconductor to the narrow-band-gap one and be accumulated in one semiconductor and subsequently recombine. In type II heterojunction, both the valence and conduction bands of one semiconductor are higher in energy than those of another semiconductor, and the transfer of electrons from a semiconductor with higher conduction band level (SC 1 in Figure 2b) to a semiconductor with lower conduction band level (SC 2 in Figure 2b) is formed, while holes migrate from lower valence band in SC 2 to higher valence band in SC 1 (Figure 2b). In this case, electrons and holes are accumulated in different semiconductors, and their lifetime is thus increased.
As a rule, charge carrier separation mechanisms in composite photocatalysts composed of g-C3N4 and another semiconductor are based on type II heterojunction or direct Z-scheme. Later, S-scheme heterojunction was proposed[33]. The direct Z-scheme transfer mechanism occurs between two semiconductors the energy structure of which is similar to that described above for type II heterojunction. However, in this case, an electron with a low reduction potential (in SC 2) recombines with a hole that has a low oxidation potential (in SC 1); in comparison with type II heterojunction, this gives rise to charge carriers with a higher redox potential. The separation of charge carriers between two semiconductors according to the S-scheme is similar to the direct Z-scheme; however, the S-scheme takes into account the semiconductor band bending caused by alignment of their Fermi levels and generation of the built-in electric field in the photocatalyst[34]. As a rule, Z-scheme is implemented by two n-type and p-type semiconductors, while S-scheme is implemented by n-type semiconductors, considered as an oxidation photocatalyst and reduction photocatalyst (see Figure 2)[3, 35, 36]. Both Z-scheme and S-scheme can be either direct (Figure 2d) or indirect (Figure 2e); in the latter case, compounds with high electrical conductivity, e.g., metals or some carbon materials such as graphene, serve as mediators[37]. It is important that implementation of either Z- or S-scheme in a composite photocatalyst not only increases the efficiency of separation of the electron – hole pairs, but also maximizes the redox capacity of charge carriers.
One more popular method for increasing the lifetime of photogenerated charge carriers is deposition of metals on the semiconductor surface. The Schottky junction is formed at the metal-semiconductor interface, and electrons from the conduction band of the semiconductor move to Fermi level of metal, which leads to alignment of the metal and semiconductor Fermi levels and bending of semiconductor bands near the interface.
Apart from the lifetime of photogenerated charge carriers, another important characteristic of semiconductor photocatalysts is the size of the optical band gap (below referred to as band gap), which determines the minimum energy of light quantum necessary for generation of electron – hole pairs (Figure 3)[38]. As a rule, the band gap energy is derived from the diffuse reflectance spectroscopy by the method proposed by Jan Tauc[39]. First, the absorption coefficient \( F(R) \) is found using the Kubelka – Munk equation:
where \( R \) is the reflection factor of the sample determined from the diffuse reflectance spectroscopy data.
Using the Tauc equation, the dependence of the absorption coefficient \( F(R) \) on the photon energy is found:
where \( h \) is the Planck constant, \( \nu \) is the photon frequency, \( E_g \) is the band gap energy, \( B \) is a constant, \( \gamma \) is the factor equal to 1/2 for direct junctions and to 2 for indirect junctions.
The intersection of the tangent to the Tauc plot with the abscissa gives the band gap energy of the semiconductor. It is noteworthy that this method is suitable for determining the band gap energy of unmodified semiconductors. Determining the band gap energy for composite materials, especially in the case of different types of electron transfer (direct/indirect) in semiconductors gives, most often, incorrect results. Nevertheless, in many publications, analysis of band gap changes based on diffuse reflectance spectroscopy is often performed for composite materials. In this case, the spectrum of the photocatalyst is usually a linear combination of the spectra of independet components, except the cases where quantum size effects or localized surface plasmon resonance influence the optical properties of materials. In some cases, the band gap of modified semiconductors can be estimated by drawing the tangent to the region called Urbach tail in the diffuse reflectance spectra. Then the intersection of this tangent with the tangent to the Tauc plot gives a correct band gap energy for a single component of a photocatalyst[40].
It is known that graphitic carbon nitride can behave as either p-type or n-type semiconductor depending on the external effects such as the applied voltage and redox potential of a reagent[41-45]. For example, g-C3N4 behaves as a p-type semiconductor when a negative voltage is applied and as an n-type semiconductor when the voltage is positive; therefore, it can be considered as an amphoteric semiconductor[42, 46, 47]. The amphoteric semiconductor properties also depend on the g-C3N4 bulk structure and surface functionalization[48]. Hence, the charge carrier transfer mechanism in composite photocatalysts composed of g-C3N4 and a semiconductor with a high potential of photogenerated holes can be considered as a Z-scheme or S-scheme, as described below.
Traditionally, g-C3N4 is obtained by heat treatment of various nitrogen-containing precursors such as melamine, dicyandiamide, and urea[49-51], but, most often, this method does not provide a material with a high specific surface area. In recent years, numerous new approaches to the synthesis of g-C3N4 have been proposed providing the possibility of varying the particle size, pore volume and specific surface area of the resulting material[52]. The methods of synthesis of g-C3N4 are comprehensively addressed in the literature[22, 53-57]; therefore, they are not discussed here.
This review is focused on g-C3N4 heterostructures with other materials used for photocatalytic CO2 reduction. It is noteworthy that there are a few published reviews dealing with modification of g-C3N4 and fabrication of heterostructures for the photocatalytic reduction of CO2[58-64] considering photocatalysts in the light of charge carrier transfer mechanisms or the effect of methods of synthesis on the photocatalytic properties. There are also reviews on using g-C3N4-based systems for other photocatalytic reactions (hydrogen evolution, decomposition of dyes, water treatment, etc.)[54, 65, 66]. A widely used photocatalyst is g-C3N4 with platinum deposited on its surface, which is obtained by photoreduction of H2PtCl6 in a solution of an electron donor, most often, triethanolamine or by reduction with a solution of NaBH4[67, 68]. Modification of semiconductors with transition metals to enhance the photocatalytic activity has also been studied in detail and presented in the literature[69-71].
This review is devoted to the most recent advances in the field of g-C3N4-based heterostructures for photocatalytic CO2 reduction. The attention is focused on the materials used and the most likely mechanism of charge carrier transfer between the semiconductors. In addition, a distinctive feature of this review is comparison of the photocatalyst activity in terms of the overall rate of consumption of photogenerated electrons, which makes it possible to compare the activities of photocatalysts with different selectivities to CO2 reduction products. The review addresses the most widely used and promising systems containing metal oxides and sulfides, two-dimensional metal carbides (MXenes) and carbon materials. Since in some cases the literature provides information about different possible heterojunctions for the same type of heterostructure, the role of the type of charge carrier separation mechanism is also analyzed in each Section.
2. g-C3N4-based heterostructures with metal oxides
2.1. Heterostructures with titanium dioxide
Titanium dioxide is one of the most popular photocatalysts used both for CO2 reduction and for other photocatalytic processes, which is due to the low cost, stability and low toxicity of TiO2[72-74]. However, the use of TiO2-based photocatalysts is limited by the large band gap (~3.2 eV), since only UV photons have sufficient energy to induce the photoexcitation in TiO2[12, 75]. As a consequence, TiO2 has low activity under the sunlight, in which the fraction of ultraviolet radiation is much smaller than that of the visible radiation. To increase the response of TiO2-based photocatalysts to visible light, TiO2 is modified with narrow-band-gap semiconductors, for example, g-C3N4[76].
Titanium dioxide is known to have three stable crystalline phases — anatase, rutile, and brookite (Figure 4)[21, 77-79]. As a rule, the first two of them are used in photocatalytic studies; anatase is considered to be more active than rutile, because of the higher reduction potential of electrons and the ability to form hole traps[80, 81], which is attributable to different predominant orientations of the crystallite surfaces in rutile and anatase. In the case of anatase, the crystallite surface is enriched with {101} and {001} faces, while in the case of rutile, {110}, {100} and {101} faces predominate[82]. It is known that the {001} face is more reactive in photocatalytic reactions[83, 84]. Meanwhile, rutile has a narrower band gap than anatase and, hence, it can be used for reactions under irradiation at longer wavelengths[85]. The conduction band minimum in TiO2 is approximately –0.2 V (vs. NHE at pH = 7)[21], which is insufficient for CO2 reduction, since the formation potential of most products is more negative [see Eqns (1) – (5)]. However, the conduction band minimum in g-C3N4 is approximately –1.20 V (vs NHE at pH = 7), which is sufficient for the formation of various organic compounds from CO2[21].
In the formation of g-C3N4-based composite photocatalysts, the particles of other components are usually distributed over the g-C3N4 surface, because it has a layered structure where the longitudinal particle size substantially exceeds the transverse size. These composite structures are commonly designated by X/g-C3N4 (X is a component of a g-C3N4-based photocatalyst), e.g., TiO2/g-C3N4. This notation is used in this review, although authors of original publications may use other designations for heterostructures.
A fairly widespread method for the synthesis of TiO2/g-C3N4 composites is physical mixing of components, according to which the components (TiO2 and g-C3N4) are synthesized separately[19, 86]. TiO2 is obtained, most often, by hydrolysis, hydrothermal or solvothermal treatment of titanium-containing precursors such as titanium alkoxides[19, 73].
Mehregan et al.[87] proposed a method for the fabrication of TiO2/g-C3N4 composite by hydrothermal treatment [hereinafter, slash (/) is used in the designations of heterostructures, while a hyphen (–) designates semiconductors doped with metal or non-metal atoms]. Titanium dioxide was prepared by the sol – gel method using titanium tetrabutoxide as the precursor, while g-C3N4 was obtained by melamine calcination followed by exfoliation to give a layered structure (Figure 5). A suspension consisting of weighed portions of TiO2 and g-C3N4 obtained in this way (in 2 : 1 w/w ratio of TiO2 to g-C3N4) and aqueous ethanol was sonicated and then placed in an autoclave and kept at 120 °C for 3 h. The CO2 reduction was carried out in the gas phase in the presence of water vapour on exposure to visible light. The authors studied the effect of light intensity on the reaction rate and showed a pronounced increase in the photocatalyst activity with as the light power density increased from 20 to 80 mW cm–2. Thus, the light intensity of 80 mW cm–2 provided the highest product formation rates, 33 and 1.4 mmol g–1 h–1 for CH4 and CH3OH, respectively. In some other publications, it is also shown that increase in the light power density increases the rates of electron and hole generation, which results in a higher photocatalyst activity[88, 89].
It should be emphasized that Evonik P25, a commercial TiO2 powder, is used most often for the synthesis of various TiO2-based photocatalysts[12]. This TiO2 powder is composed of 80% anatase and 20% rutile with an average particle size of 25 nm and has a specific surface area of 40 – 60 m2 g–1[90]. Wang et al.[91] synthesized TiO2/g-C3N4 composite photocatalysts with various component ratios by ball milling of TiO2 (anatase) with g-C3N4 pretreated with a solution of HNO3 to form a layered structure (Figure 6). Then the mixture was calcined at 400 °С for 1 h to form the composite material with TiO2 particles deposited on the g-C3N4 surface. The authors demonstrated a change in the positions of the valence and conduction bands depending on the component ratio and increase in the band gap with increasing TiO2 content. This study describes the liquid-phase СО2 reduction in which the suspension with a photocatalyst in aqueous NaOH and triethanolamine solution was placed in a reactor, and then purged with СО2 flow. The highest activity after 4 h of UV irradiation (8 W lamp) was found for the photocatalyst with TiO2 to g-C3N4 ratio of 1 : 2. The rates of СО and СН4 formation were 14 and 18 mmol g–1 h–1, respectively, which was twice as high as that for pristine g-C3N4.
Truc et al.[92] synthesized composite photocatalysts based on g-C3N4 and niobium-doped TiO2 in which the direct Z-scheme heterojunction was formed (Figure 7)[92]. The introduction of Nb into TiO2 structure leads to the formation of Ti3+ ions and the appearance of an additional energy level near the TiO2 conduction band, thus reducing the band gap size from 3.2 to 2.9 eV. The change in the band gap by introducing metal and non-metal ions into a parent substance is a common method used to shift the absorption edge of photocatalysts[93-97]. The Nb – TiO2/g-C3N4 composite photocatalysts were obtained by calcination of a mixture of Nb – TiO2 with melamine. In a series of experiments on the gas-phase photocatalytic CO2 reduction on exposure to light from two 30-W white lamps, the highest activity was detected for the photocatalyst with 1 : 1 ratio of (Nb – TiO2) to g-C3N4. Apart from CO and CH4, the reaction products were found to contain formic acid and oxygen resulting from water oxidation. The formation rates of CO, CH4, HCOOH and O2 were 420, 560, 700 and 1700 mmol g–1 h–1, respectively. The results obtained in this work demonstrate the high potential of the integrated approach to the synthesis of photocatalysts, including both doping and the formation of heterojunctions in composite materials.
To compare the activity of photocatalysts with different selectivities to reaction products, it was proposed to use the total number of electrons absorbed in the photocatalytic process per unit time and per unit mass of the photocatalyst, We, which is calculated by the equation[98]:
where WCO, WCH4, WCH3OH , and WHCOOH are the rates of formation of the products per unit mass of the photocatalyst (as a rule, mmol g–1 h–1); coefficients are determined by the number of electrons needed for the formation of this product. Methane makes the greatest contribution to We, because its formation requires more electrons than the formation of other compounds [see Eqns (1) – (5)]. Equation (8) or similar relations are convenient for calculating the apparent quantum efficiency (AQE) [Equation (9)] and are widely used in the studies of the photocatalytic CO2 reduction, although the terminology may differ[7, 98-101].
where We* is the product formation rate with allowance for the electron balance (usually μmol h–1), Nph is the photon flux from the light source calculated from the data on the irradiation power and spectrum (mmol h–1).
Unfortunately, AQE values for the photocatalytic CO2 reduction (usually not exceeding 1%) are rarely reported in the literature; therefore, comparison of the photocatalyst activity will be based on calculation of the rate We[12]. However, it is noteworthy that AQE is an important characteristic of photocatalytic systems, as it reflects the photon utilization efficiency, while the rate We depends, among other factors, on the light irradiation power. The only outstanding AQE value was repotrted by Zhang et al.[102] A composite photocatalyst based on g-C3N4 and Ag-doped TiO2 has an AQE of 2.4% in the liquid-phase CO2 reduction. It is shown that the addition of Ag promotes implementation of S-scheme heterojunctions and electron transfer from TiO2 to g-C3N4. In addition, high efficiency of CO2 reduction is attained owing to high specific surface area and defects in the g-C3N4 structure.
The data on TiO2/g-C3N4 composite systems that were reported in recent years are summarized in Table 1, which also indicates the reaction conditions and reagents added to the reactor. To carry out the photocatalytic reaction, a flow of ultrapure СО2 is usually purged through the reactor; however, the СО2 generation directly in the reactor, e.g., by the reaction of NaHCO3 and H2SO4, can also be used. Most often, the source of protons in photocatalytic CO2 reduction is water, but CH4 can also serve for this purpose. Generally, TiO2 is one of the most popular semiconductors for the synthesis of photocatalysts; therefore, there are numerous heterostructures based on TiO2. A combination of TiO2 with g-C3N4 in the TiO2/g-C3N4 composite photocatalysts gives We values reaching 270 mmol g–1 h–1 under visible light irradiation[87], while modification of the TiO2/g-C3N4 composite leads to even a more pronounced increase in the activity. As can be seen from Table 1, high We value was attained for composite system comprising Z-scheme heterojunction and synthesized from TiO2 doped with metal ions; therefore, this method appears to be most efficient.
2.2. Heterostructures with zinc oxide
Zinc oxide ZnO is widely used in various fields owing to its mechanical, electrical, optical and photocatalytic properties[113, 114]. The most thermodynamically stable ZnO phase under ambient conditions is wurtzite (Figure 8)[115, 116]. ZnO has a band gap of approximately 3.4 eV and n-type conduction, which makes it similar to TiO2 for photocatalytic applications[117]. Hence, combination of ZnO with narrow-band-gap semiconductors such as g-C3N4 is also a promising approach for increasing the photocatalytic activity similarly to TiO2/g-C3N4 composites.
A variety of methods for the preparation of ZnO/g-C3N4 composites such as hydrothermal and solvothermal synthesis, layer-by-layer deposition and other have been reported in the literature[19]. For example, Chen et al.[118] synthesized the ZnO/g-C3N4 composite photocatalyst by evaporation of a suspension consisting of a methanol solution, g-C3N4 and zinc acetate prepared in advance. The reduction involved CO2 and water vapour, which were formed inside the reactor upon the reaction between NaHCO3 and H2SO4. The We value found for the composite photocatalyst was 9.4 times higher than that for pristine g-C3N4. The use of We for comparison of the photocatalyst activities is especially important here, because the photocatalytic СО2 reduction in the presence of g-C3N4 is dominated by the formation of CO, whereas in the case of ZnO/g-C3N4 composites, the reaction almost exclusively gives СН4. The authors also studied the stability of the most active ZnO/g-C3N4 photocatalyst and demonstrated that the activity decreased by about 7% by the third cycle, which indicated a high stability of the synthesized photocatalyst.
Guo et al.[119] synthesized the ZnO@g-C3N4 composite photocatalyst with the core@shell structure by depositing g-C3N4 on porous ZnO nanosheets using two-step calcination and studied the effect of reaction temperature on the photocatalyst activity towards the CO2 reduction. A temperature rise from 150 to 200 °C resulted in a 3-fold and 2-fold increase in the rate of CH4 and CO formation, respectively. However, as the temperature was further increased to 250 °C, the formation rate of CO2 reduction products increased by only 33% compared to the rate at 200 °C. This effect may be caused by diffusion processes and adsorption – desorption equilibrium, which are affected by temperature. Since impossibility of initiation of photocatalytic processes by thermal energy has been proved in the literature both experimentally and theoretically, the change in the activity can be due only to the change in the above mentioned dark stages of the reaction[12, 120-122].
Zhu et al.[123] deposited copper nanoparticles on the ZnO/g-C3N4 materials with different contents of ZnO to be used in the photocatalytic CO2 reduction in aqueous solution under mercury lamp irradiation. The highest rates of CO, CH4 and CH3OH formation equal to 64, 41 and 93 mmol g–1 h–1, respectively, were attained by using the 3% Cu/(30% ZnO/g-C3N4) photocatalyst (The contents of the components are given in mass percent.). These values are not only markedly higher than those for pristine g-C3N4, but they are also higher than those for the 30% ZnO/g-C3N4 composite photocatalyst. The authors proposed a possible microstructure of the 3% Cu/(30% ZnO/g-C3N4) photocatalyst and a mechanism of separation of the photogenerated charge carriers (Figure 9). Presumably, the charge carrier separation mechanism implemented in the photocatalysts is similar to type II heterojunction, but occurs via copper nanoparticles, which act as not only electron traps, but also as sources of electrons, causing a significant increase in the photocatalytic reaction rate.
Data on some ZnO/g-C3N4 systems are summarized in Table 2. Currently, ZnO has been less explored for photocatalytic applications than TiO2; hence, relatively few data on heterostructures based on ZnO composites with g-C3N4 have been reported in the literature. It is important that the activity of ZnO/g-C3N4 photocatalysts in the CO2 reduction is at the level of TiO2/g-C3N4 composite activity. Moreover, the We value of 1010 mmol g–1 h–1 for Cu/ZnO/g-C3N4 is especially notable, because three СО2 reduction products, CO, CH4 and CH3OH, are formed at high rates in this case. Most types of heterojunctions present in the ZnO/g-C3N4 photocatalysts reported in the literature correspond to type II. However, the highest We value was attained for a three-component composite photocatalyst with a complex of heterojunctions.
2.3. Heterostructures with cerium dioxide
Cerium dioxide CeO2 is an n-type semiconductor with a wide band gap (2.8 – 3.1 eV) and the fluorite structure[128, 129]. An important feature of this compound is the high proneness of Ce4+ cations to be reduced to Ce3+[130]. This change in the oxidation state results in a change in the stoichiometry and formation of oxygen vacancies, which are known to enhance the absorption of visible light and also act as photoinduced charge carrier traps or as adsorption sites (Figure 10)[131, 132].
Liang et al.[133] investigated hollow g-C3N4@CeO2 photocatalysts for CO2 reduction under irradiation with a xenon lamp with a 420 nm cut-off filter (l > 420 nm). The heat treatment of the composite photocatalyst in a hydrogen atmosphere resulted in the formation of oxygen vacancies and partial Ce4+ reduction to Ce3+. The highest rates of CH4, CH3OH and CO formation were attained in the presence of the g-C3N4@49.7%CeO2 photocatalyst and amounted to 1.2, 1.7 and 5.6 mmol g–1 h–1, respectively, which exceeds these values for single g-C3N4 and CeO2 catalysts. The synergistic effect is caused by the formation of type II heterojunction between the two semiconductors and by the large number of oxygen vacancies in CeO2. The Ce4+ cations can trap photoinduced electrons, whereas the Ce3+ cations apparently provide the formation of the CO2 – radical anion, which is an intermediate of CO2 reduction[134]. Moreover, the hollow structure of the photoсatalyst increases the light utilization efficiency due to multiple reflections[135].
Wang et al.[136] synthesized a series of CeO2/g-C3N4 composite photocatalysts with a built-in electric field. The electric field formation was confirmed by density functional theory (DFT) calculations. The calculation results indicate that electrons are accumulated on the g-C3N4 surface, while holes are concentrated on CeO2. This gives rise to a built-in electric field directed from CeO2 to g-C3N4, which promotes the transfer of photogenerated charge carriers according to the S-scheme. The highest product formation rates attained on the 1.75% CeO2/g-C3N4 photocatalyst were 0.56 and 15 mmol g–1 h–1 for CO and CH4, respectively, which is almost 20 times higher than those attained with single g-C3N4 or CeO2.
Li et al.[137] reported a multistep hydrothermal synthesis of the CeO2/g-C3N4 composite photocatalyst; then partially reduced graphene oxide (rGO) was deposited on the composite surface. Partially reduced graphene oxide is a graphene-like material with specific structural defects and oxidized groups on the surface[138]. The combination of these characteristics gives rise to a two-dimensional material with a large specific surface area, high electron mobility and chemical stability, which makes it a promising component for the synthesis of composite photocatalysts[139]. The photocatalytic CO2 reduction was carried out in a suspension consisting of an alkaline solution of TEOA and a photocatalyst under xenon lamp irradiation. The CO and CH4 formation rates were 63 and 33 mmol g–1 h–1 for rGO/CeO2/g-C3N4 vs 15 and 5.2 mmol g–1 h–1 for g-C3N4. The results of DFT calculations suggest the formation of a built-in electric field in the composite photocatalyst caused by changes in the Fermi levels, similarly to what was described by Li et al.[137]. In the resulting multicomponent heterostructure, the formation of S-scheme heterojunction for electrons was suggested.
Data on the CeO2/g-C3N4 systems are summarized in Table 3. The use of CeO2 in photocatalytic studies is primarily due to the variable stoichiometry. The highest We values for CeO2-based composite photocatalysts were obtained for heterostructures in which the charge carrier separation mechanism corresponds to the S-scheme and which are used for liquid-phase CO2 reduction. As can be seen from Table 3, the formation of S-scheme hetero-junction provides the highest activity of CeO2/g-C3N4-based photocatalysts. However, the activity of photocatalysts of this type is usually lower than that of TiO2/g-C3N4 or ZnO/g-C3N4. Apparently, CeO2 may be a promising material for the synthesis of photocatalysts for СО2 reduction; however, additional studies along this line are needed.
2.4. Heterostructures with iron oxide α-Fe2O3
The iron oxide α-Fe2O3 (hematite) is a readily available, thermodynamically stable and environmentally benign narrow-band-gap n-type semiconductor[146-149]. However, the narrow band gap (2.2 eV) not only enhances the visible light absorption, but also markedly decreases the lifetime of photogenerated electron – hole pairs[150]. Moreover, the energy level of the bottom of the conduction band hampers the use of this material in reduction reactions due to the low potential of photogenerated electrons[19, 151]. Thus, α-Fe2O3 can be used in the photocatalytic CO2 reduction only upon the formation of heterostructures with other semiconductors. In this respect, g-C3N4 can act as a semiconductor with an appropriate level of the conduction band minimum and with wider band gap compared to that of α-Fe2O3.
Guo et al.[152] used the hydrothermal method to prepare the α-Fe2O3/g-C3N4 composite for the photocatalytic CO2 reduction to CH3OH in water under xenon lamp irradiation with a 420 nm cut-off filter. The highest CH3OH formation rate is 5.6 mmol g–1 h–1, which is almost three times higher than that for pristine g-C3N4 (1.9 mmol g–1 h–1). This increase is attributable to the formation of direct Z-scheme, which prevents recombination of photogenerated charge carriers and promotes the generation of electrons with a high reduction potential.
Duan and Mei[153] synthesized the α-Fe2O3/g-C3N4 photocatalyst by hydrothermal method from a colloidal solution containing both components (Figure 11). The CO2 reduction reaction was conducted in an aqueous solution of DMF and TEOA under irradiation with a 60 W white light emitting diode (LED). The major reaction product was CH3OH formed at a high rate of 74 mmol g–1 h–1. The solvent effect on the reaction rate was investigated by replacing DMF with acetonitrile, but the photocatalyst activity significantly decreased, indicating that the use of DMF in the photocatalytic CO2 reduction may be promising for α-Fe2O3/g-C3N4 photocatalysts (but not necessarily for other ones[154]). It is worth noting that under the light irradiation, TEOA can be converted in the reaction medium to give carbon-containing products, which can affect the photocatalytic reaction rate[155-158]. This effect was ruled out by conducting an experiment with 13CO2; this confirmed that CO2 was the only source of CH3OH.
Padervand et al.[159] synthesized a complex composite photocatalyst, K4Nb6O17/Fe3N/α-Fe2O3/C3N4, by one-step thermal pyrolysis of the precursor. The photocatalyst combines the properties of three semiconductors with different positions of bands and magnetic properties of Fe3N, which produces a highly efficient system for photocatalytic reactions. The highest product formation rates in the gas-phase CO2 reduction under visible light irradiation were 7.0 and 1.3 mmol g–1 h–1 for CO and CH4, respectively. The authors also proposed an electron transfer mechanism: since K4Nb6O17 has a wider band gap with a very low top of valence band, type I heterojunction with α-Fe2O3 can be formed simultaneously with S-scheme heterojunction between α-Fe2O3 and g-C3N4. Moreover, Fe3N acts as an electron trap, similarly to metals. These features explain the high CO2 conversion upon photocatalyst irradiation with visible light.
Data on α-Fe2O3/g-C3N4 systems reported recently are summarized in Table 4. The highest We value of 440 mmol g–1 h–1 was found for the reaction in an aqueous solution of DMF + TEOA, whereas standard conditions of CO2 reduction in aqueous solutions or in the presence of water vapour resulted in a lower rate, even when complex heterostructures were used as photocatalysts. Thus, the formation of composite photocatalysts consisting of g-C3N4 and narrow-band-gap semiconductors such as α-Fe2O3 is not a promising trend. In this case, high rates of product formation are not attained, probably because of the low lifetime of photogenerated charge carriers caused by the narrow band gaps of both components.
3. Heterostructures with metal sulfides
3.1. Heterostructures with cadmium sulfide
Cadmium sulfide CdS is a semiconductor material widely used for photocatalytic CO2 reduction and many other photocatalytic reactions, because it has a narrow band gap (~2.4 eV) and appropriate arrangement of the valence and conduction bands[162-165]. Meanwhile, the photocatalytic application of CdS is limited not only by recombination of electron – hole pairs, but also by photocorrosion caused by the oxidation of sulfide ions by photogenerated holes[166-169]. An effective method to overcome these drawbacks is to fabricate composite photocatalysts based on CdS and other semiconductors.
Vu et al.[170] synthesized the CdS/g-C3N4 composite and studied it in the photocatalytic CO2 reduction under irradiation with a solar simulator (100 mW cm–2); the photocatalyst was suspended in a solution containing acetonitrile, TEOA, water and [Co(bpy)3]Cl2. An aqueous solution of acetonitrile was used as a solvent, TEOA served as an electron donor and the organometallic complex acted as a co-catalyst. It is noteworthy that no CO2 reduction products were detected in the absence of TEOA and the Co complex. Carbon monoxide was formed as the major product of CO2 reduction, CH4 was detected in a small amount, and H2 was formed as a by-product. The rate of СО evolution was 240 mmol g–1 h–1, which was almost four times higher than that with pristine g-C3N4. The selectivity to СО was 73%. The photocorrosion of CdS was inhibited via migration of photogenerated holes to g-C3N4: study of the photocatalyst stability indicated no loss of activity after four reaction cycles. The synergistic effect of CdS/g-C3N4 heterostructure is attributable to implementation of the direct Z-scheme with a bridging C – S – Cd bond at the interface, which results in increasing rate of transfer and separation of photogenerated charge carriers (Figure 12).
Guo et al.[171] synthesized a photocatalyst based on g-C3N4 and Zn0.2Cd0.8S nanoparticles using a combination of ultrasonic treatment and hydrothermal method (Figure 13). The ZnxCd1–xS solid solutions are known as photocatalysts that are activated under visible light and have a tunable band structure, which can be controlled by varying the Zn : Cd ratio, as in other solid solutions[172-175]. The photocatalytic activity in the CO2 reduction was measured in an aqueous suspension of the photocatalyst at 80 °C under irradiation with a xenon lamp with a 420 nm cut-off filter. Methanol was the only detected product, which formed at a rate of 12 mmol g–1 h–1; this was higher than the methanol formation rate in the reaction performed using single components of the photocatalyst. Stability testing showed a decrease in the activity by only 7% after 28 h of the reaction, which indicated successful inhibition of photocorrosion. The authors suggested that separation of the photogenerated charge carriers in this photocatalyst corresponds to type II heterojunction, which promotes increase in both stability and activity.
Data on some CdS/g-C3N4-based systems are summarized in Table 5. Since CdS is also a narrow-band-gap semiconductor, its combination with g-C3N4 usually does not provide high rates of CO2 reduction. To attain high activity, additional modification or more complex reaction system is required. In particular, the two highest We values were found for systems using m-CdS/g-C3N4 photocatalyst dispersed in a solution, whereas We observed for the gas-phase СО2 reduction in the presence of (Au/ZnxCd1–xS)@g-C3N4 was only 7.9 mmol g–1 h–1.
3.2. Heterostructures with tin sulfide
Tin disulfide SnS2 is a non-toxic semiconductor with a narrow band gap (~2.2 eV); therefore, it may be of interest for photocatalytic studies as a combination with wider-band-gap semiconductors[179-184].
Wang et al.[185] synthesized SnS2/g-C3N4 composite photocatalysts with type II heterojunction by using self-assembly based on the electrostatic interactions between the components in an ethanol solution. The prepared photocatalysts with different SnS2 contents were tested in the photocatalytic CO2 reduction under xenon lamp irradiation. The highest rate of formation of the major product (CO) was 0.64 mmol g–1 h–1 in the presence of 60%SnS2/g-C3N4, which is much higher than the rates attained using single g-C3N4 or SnS2. The stability testing carried out for the most active sample showed that the activity remained almost unchanged after four cycles of the photocatalytic reaction. Since the results of X-ray diffraction and X-ray photoelectron spectroscopy did not show any significant changes either, it can be assumed that the synthesized photocatalyst has a high photocatalytic stability. Study of the charge carrier transfer mechanism provided the conclusion that type II heterojunction is formed between g-C3N4 and SnS2. This increases the charge carrier separation rate, but, simultaneously, it also leads to a decrease in the redox potentials of photogenerated electrons and holes.
Yin et al.[186] obtained the SnS2/g-C3N4 composite photocatalyst by a two-step method comprising sonication and hydrothermal synthesis followed by photodeposition of Au nanoparticles. The resulting photocatalyst was studied in CO2 reduction in an aqueous solution of TEOA under irradiation with a xenon lamp. The major reaction products were CO and CH4, with their formation rates being 94 and 75 mmol g–1 h–1, respectively, while g-C3N4 and SnS2 taken separately had lower activity. The synergistic effect is due to two factors. First, both the hydrophilicity and CO2 adsorption increase in the series g-C3N4 < SnS2 < SnS2/g-C3N4 < SnS2/Au/g-C3N4, which leads to increasing adsorption of the reactants on the photocatalyst surface. Second, it is assumed that gold nanoparticles act as electron mediators for the Z-scheme implemented in the composite photocatalyst, which facilitates the electron transfer and increases the rate of charge carrier separation.
SnS2 is not the only tin sulfide that is used as a photocatalyst. For example, Huo et al.[187] reported hydrothermal synthesis of a composite photocatalyst consisting of porous g-C3N4 and Sn2S3 modified with diethylenetriamine (DETA) (Figure 14). The CO2 reduction experiment was carried out in the gas phase in which CO2 and water were formed upon the reaction of NaHCO3 with HCl. A xenon lamp with a >420 nm cut-off filter was used as the light source. The major reaction products were CH4 and CH3OH, with the rates of their formation being 4.9 and 1.5 mmol g–1 h–1, respectively; this is higher than the activities of the initial g-C3N4 and Sn2S3-DETA. The replacement of H2SO4 by HCl did not result in any noticeable change in the reaction rate, which implies that the acid is not involved in the photocatalytic reaction. It is assumed that the synergistic effect is due to implementation of direct Z-scheme in the synthesized composite photocatalyst with a built-in electric field.
The data on the systems based on tin sulfides and g-C3N4 are summarized in Table 6. Despite the fact that the tin sulfide band gap is narrower than that of g-C3N4, tin sulfides (II, III, IV) are also used to form heterostructures. Apparently, SnS is potentially the most appropriate photocatalyst in this series for the photocatalytic CO2 reduction, because the highest We value of 980 mmol g–1 h–1 was observed for SnS/g-C3N4 system in the gas-phase reduction of CO2 under irradiation with a solar simulator. For comparison, the SnS2/g-C3N4 system modified by gold nanoparticles, which was tested in the liquid-phase reduction of СО2 under irradiation with full-spectrum xenon lamp, provided We of 790 mmol g–1 h–1. Note that tin sulfides are not widely used for the synthesis of heterostructures with g-C3N4, and there are few publications describing the use of tin sulfides as photocatalytic materials for CO2 reduction, although SnS-based photocatalysts can potentially exhibit a fairly high activity in other reactions, for example, decomposition of dyes[192].
4. Heterostructures with 2D materials
4.1. Heterostructures with MXenes
MXenes are a new class of 2D metal carbides, nitrides or carbonitrides discovered in 2011[193]. As a rule, MXenes are obtained from MAX phases by removing layers of A element (usually Al) with a potent etching reagent containing F– anions (Figure 15)[193-198]. Different methods of synthesis result in the formation of different surface functional groups (e.g., –O, –F, –OH and –Cl). The general formula of MXenes can be written as Mn+1XnTx, where M is transition metal, X is C or N, and T is a functional group. MXenes have a number of remarkable properties such as high electrical conductivity, hydrophilicity and ordered layered structure, also, the composition of functional groups on their surface can be controlled[197, 198]. MXenes have already proved to possess a high potential for many applications including photocatalysis and are considered as promising materials for photocatalytic CO2 reduction, especially in combination with other semiconductors forming heterostructures. The electronic structure of MXenes is characterized, most often, by the absence of band gap and high electron work function; therefore, they can be used as co-catalysts to form Schottky junction at the semiconductor – MXene interface, similarly to heterostructures based on transition metals and semiconductors.[199]
Li et al.[200] developed mesoporous Ti3C2Tx/g-C3N4 photocatalysts for CO2 reduction under irradiation with a xenon lamp (Figure 16). The MXene phase was prepared by a widely used method involving etching of Ti3AlC2 with hydrofluoric acid to remove Al layers. The reduction of CO2 in the presence of Ti3C2Tx/g-C3N4 gave CO and CH4 as the major products, with the formation rates being 4.0 mmol g–1 h–1 for CO and 2.2 mmol g–1 h–1 for CH4; these values attained with mesoporous g-C3N4 were 3.1 and 0.88 mmol g–1 h–1, respectively. This effect is attributable to higher specific surface area, larger number of defects, higher electron transfer rate and, consequently, lower recombination rate of photogenerated charge carriers.
М.Tahir and B.Tahir[201] synthesized a composite material consisting of g-C3N4 and layered bentonite clay (Bt), on which Ti3C2 particles were then deposited using the ultrasonic self-assembly technique. Bentonite mainly consists of smectite minerals, particularly montmorillonite (usually Ca montmorillonite and Na montmorillonite)[202], has a columnar multilayer structure and can significantly enhance CO2 adsorption and charge carrier separation rate owing to bentinite surface properties and the presence of metal cations, which increase the photocatalytic activity (Figure 17)[202, 203]. Indeed, the photocatalytic experiments on CO2 reduction demonstrate that the Ti3C2/g-C3N4/Bt ternary composite provides a much higher rates of formation of the major products (CO and CH4), especially CH4. It is presumed that the increase in the selectivity to CH4 is caused by the efficient heterotransfer of charge carriers between the phases, which promotes the eight-electron reduction of CO2 to СН4. The effect of a sacrificial agent is also investigated, and it is found that the addition of acetic acid increases the rate of CH4 formation by a factor of 4.2. CH3COOH acts as an electron donor, decreases the charge carrier recombination rate and serves as a source of H2, which is a more thermodynamically favourable reagent for CO2 reduction than water[203-207].
Studies dealing with composite photocatalysts for СО2 reduction based on other MXenes, apart from Ti3C2, have also been reported. For example, Madi et al.[208] synthesized the V2C/g-C3N4 photocatalyst by physical mixing of the components and sonication. The activity of the resulting photocatalyst in the gas-phase reduction of CO2 under irradiation with a 35 W xenon lamp was considerably higher than the activity of g-C3N4 and somewhat higher than the activity of V2AlC/g-C3N4. The authors attributed this synergistic effect to high electronic conductivity, which is one of the main benefits of V2C and to the formation of Schottky junction between g-C3N4 and V2C, which promotes the separation of photogenerated charge carriers[209-213]. A drawback of the composite is low stability: the photocatalyst activity decreases with every irradiation cycle, with the decrease reaching 40% by the end of the third cycle.
Data on the MXene/g-C3N4-based systems are summarized in Table 7. Analysis shows that, unfortunately, modification of g-C3N4 with Ti3C2 does not lead to a significant increase in the activity. It is noteworthy that data on the gas-phase CO2 reduction giving CO and CH4 as the major products are mainly presented in the literature. An outstanding We value of 8400 mmol g–1 h–1 was attained for the system containing bentonite clay under irradiation with a xenon lamp and with addition of acetic acid to the reaction system. A relatively simple V2C/g-C3N4 heterostructure had We of 490 mmol g–1 h–1, which far exceeded the activity of most Ti3C2/g-C3N4 systems. It can be concluded that MXenes have a great potential as co-catalysts for photocatalytic CO2 reduction and that intensive research along this line is needed.
4.2. Heterostructures with partially reduced graphene oxide
As mentioned above, partially reduced graphene oxide (rGO) is a promising material for photocatalysis due to its excellent conductivity and mechanical and optical properties combined with ready availability and the ease of synthesis from graphene oxide (GO)[138]. Moreover, a change in the reduction conditions makes it possible to obtain rGO samples that markedly differ in properties[219]. A traditional method for rGO production is the modified Hummers method, in which graphite is chemically oxidized and then exfoliated to produce graphene oxide[220]. In this stage, oxygenated functional groups appear in the graphene layers (Figure 18)[221]. During the subsequent reduction, some of these groups are removed, and the degree of reduction varies depending on the conditions[222]. It is noteworthy that the production of graphene oxide is accompanied by not only the formation of numerous oxygenated groups on the surface, but also by disruption of the conjugated structure of graphene, which provides for high mobility of charge carriers. The reduction of graphene oxide leads to the partial removal of oxygenated groups and restores the conjugated structure[223].
Li et al.[224] performed a two-step process consisting of calcination and hydrothermal treatment and thus synthesized a composite photocatalyst by combining g-C3N4 and rGO with pre-treated multi-walled carbon nanotubes (P-MWNT)[224]. The photocatalytic CO2 reduction was carried out under xenon lamp irradiation with a 420 nm cut-off filter in a TEOA and acetonitrile solution. The highest rates of CO and CH4 formation were 180 and 120 mmol g–1 h–1, respectively. The authors proposed a possible structure of the photocatalyst in which carbon nanotubes act as a mediator between g-C3N4 and rGO and increase the rate of electron transfer. Presumably, photogenerated electrons migrate from g-C3N4 to rGO (either directly or via P-MWNT) as a result of generation of a built-in electric field, which considerably increases the efficiency of separation of photogenerated charge carriers[225, 226].
Bafaqeer et al.[227] used the three-component rGO-bridged ZnV2O6/g-C3N4 photocatalyst for the photocatalytic conversion of CO2 to CH3OH in water. For experiments, the authors designed an externally reflected photoreactor to increase the efficiency of photon energy utilization. As a result, the highest CH3OH formation rate on exposure to a xenon lamp was 630 mmol g–1 h–1. Meanwhile, the CH3OH formation rate in a reactor without external reflection was about 520 mmol g–1 h–1. Thus, even without the use of reflected light energy, the obtained photocatalyst activity is quite high, which is attributable to implementation of the S-scheme between two narrow-band-gap semiconductors and to participation of rGO as a mediator for efficient photogenerated charge carrier separation.
Data on the photocatalysts based on rGO/g-C3N4 reported in recent years are summarized in Table 8.
Only ternary systems, mainly containing some type of vanadate or layered double hydroxide (LDH) as the third component, are considered here, because in the case of binary rGO/g-C3N4 photocatalysts, high СО2 reduction rates are not attained. Layered double hydroxides are a group of layered solids formed by different-valence metal ions and hydroxide ions, which possess a lot of unique properties such as possibility of surface functionalization, intercalation of anions and high chemical stability; this makes LDHs promising photocatalytic materials[233-235]. Most experiments on СО2 reduction catalyzed by rGO/g-C3N4-based photocatalysts were carried out in the liquid phase and the highest We values were found for ZnV2O6/rGO/g-C3N4 systems with Z-scheme charge transfer.
5. Conclusion
Development of g-C3N4-based photocatalysts for СО2 reduction has been a significant research subject in recent years, which is confirmed by the steadily increasing number of relevant publications. The high interest of researchers in the g-C3N4-based photocatalysts is caused by unique properties of g-C3N4, mostly, narrow band gap, which allows activation by visible light. However, narrow-band-gap semiconductors are characterized by high recombination rate of photogenerated charge carriers. Other drawbacks of unmodified g-C3N4 are low adsorption capacity towards CO2 and, as a rule, low specific surface area, which result in moderate rate of CO2 reduction. Modification of g-C3N4 mitigates the effect of these drawbacks on the photocatalytic process; the fabrication of heterostructures with other semiconductors represents the most popular modification method, as it allows for the control of the properties of photocatalysts and the subsequent increase in the CO2 reduction rate.
Currently g-C3N4 heterostructures with metal oxides and sulfides, MXenes, and rGO are the most studied composite photocatalysts based on g-C3N4. Among these materials, TiO2 is mostly studied, because it is a traditional material for many photocatalytic applications owing to its ready availability, lack of toxicity and stability. One more promising oxide semiconductor is CeO2 owing to its unique feature, that is, change in the cerium oxidation state, giving rise to electron traps and additional adsorption sites. MXenes are a new class of 2D compounds with promising properties for the formation of heterostructures and photocatalytic applications. Various approaches to MXene synthesis make it possible to vary their surface properties, which opens up the way for development of a broad range of photocatalysts. Partially reduced graphene oxide rGO has also gained popularity in recent years, and a great potential of rGO for the use in photocatalytic CO2 reduction systems has already been shown.
It is worth mentioning that comparison of the current studies on the photocatalytic CO2 reduction is difficult because no unified experimental methodology has yet been developed. Studies of similar photocatalysts under similar reaction conditions by different research groups often result in significant differences not only in the rates of product formation, but also in the set of products, which may probably be caused by incomplete removal of organic impurities from the reaction medium or by low level of experimentation. Nevertheless, even though establishing of a strict correlation is problematic, it is still possible to determine the average productivity of particular systems to identify the most promising photocatalysts. The results considered in the review provide the conclusion that TiO2 is most promising among the traditional materials used in photocatalysis for the formation of heterostructures with g-C3N4; this provides We values at the level of 200 – 600 mmol g–1 h–1, and in some cases, up to 6700 mmol g–1 h–1. In addition, there is a persistent trend towards the use of 2D materials such as MXenes and rGO for the formation of heterostructures. Indeed, in some studies, fairly high CO2 reduction rates have already been attained by using Ti3C2 or rGO to modify g-C3N4. The major products formed upon СО2 reduction catalyzed by heterostructures based on traditional semiconductors and those based on new 2D materials are CO and CH4. However, in some cases, outstanding rates of CH3OH formation have been achieved using rGO.
Thus, data on the activity of photocatalysts reported in the literature indicate that practical implementation of the photocatalytic CO2 reduction requires much more active systems and, probably, the main trend of research in the photocatalytic CO2 reduction for the next decade will be the search for and development of composite photocatalysts based on g-C3N4 combined with various 2D semiconductor materials and with materials (e.g., bentonite) that may significantly improve the textural properties of composites.
6. List of acronyms
AQE — apparent quantum efficiency,
bpy — bipyridine,
CB — conduction band,
DETA — diethylenetriamine,
DMF — dimethylformamide,
g-C3N4 — graphitic carbon nitride,
GO — graphene oxide,
GP — gas phase,
LDH — layered double hydroxide,
LP — liquid phase,
m — mesoporous,
MXene — a class of two-dimensional metal carbides, nitrides or carbonitrides,
NHE — normal hydrogen electrode,
P-MWNT — pre-treated multi-walled carbon nanotubes,
PNS — porous nanosheets,
rGO — partially reduced graphene oxide,
SC — semiconductor,
TEOA — triethanolamine,
VB — valence band,
We — total number of electrons consumed in a photocatalytic process per unit reaction time and per unit photocatalyst mass.
References
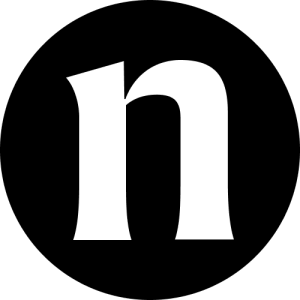
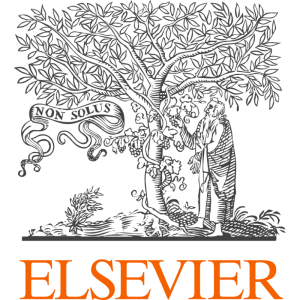
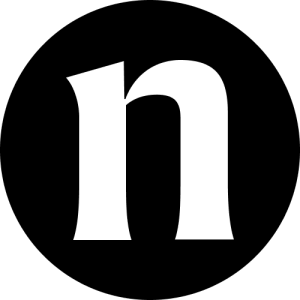
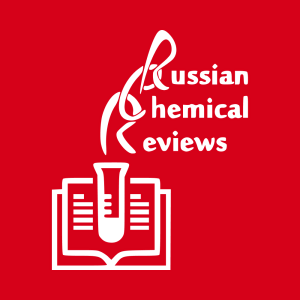
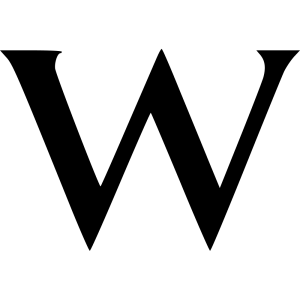
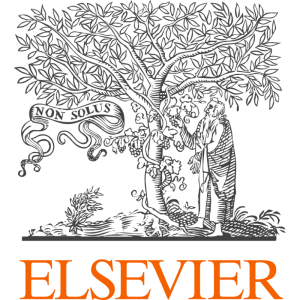
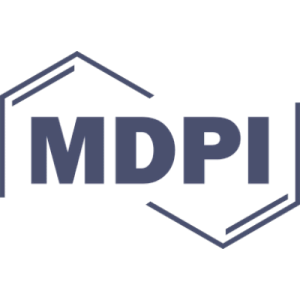
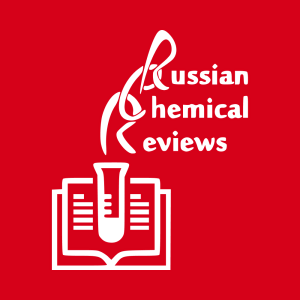
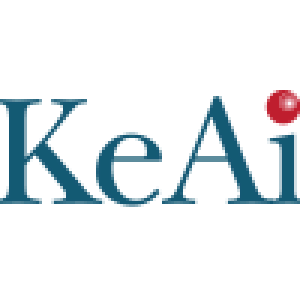
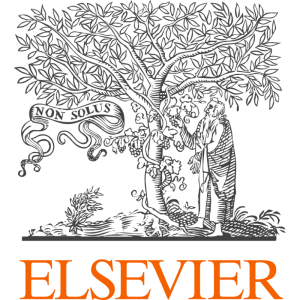
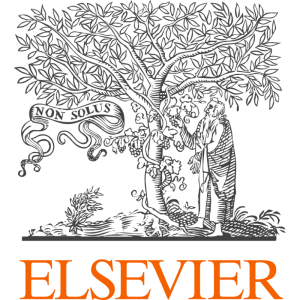
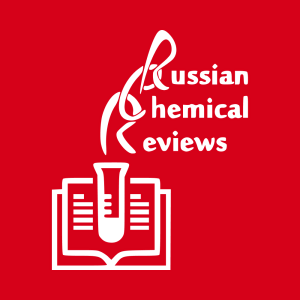
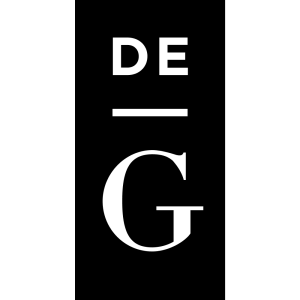
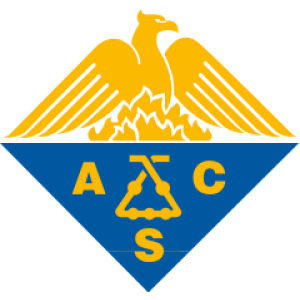
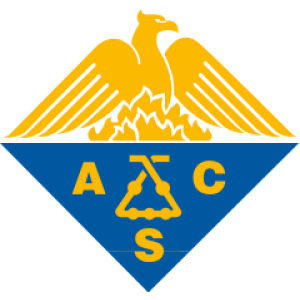
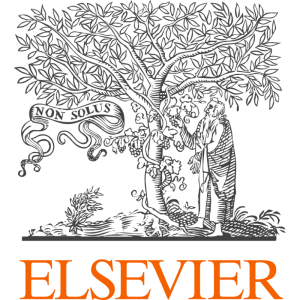
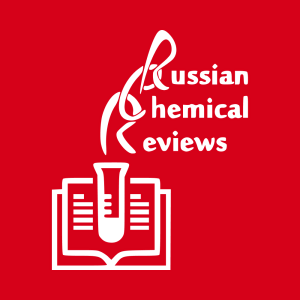
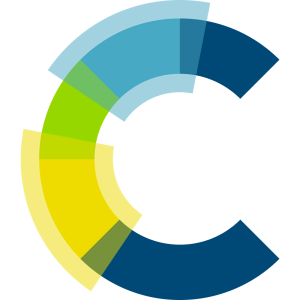
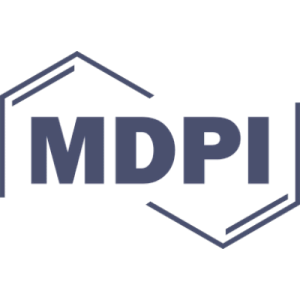
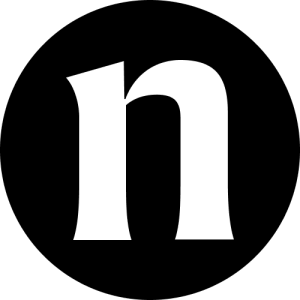
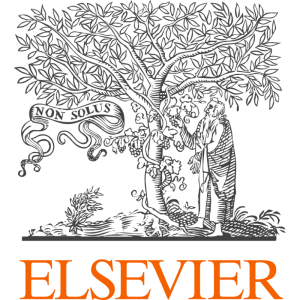
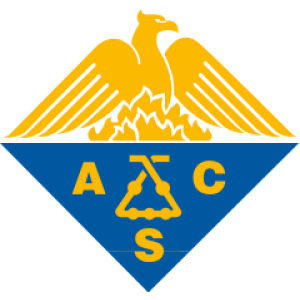
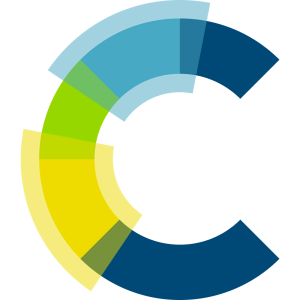
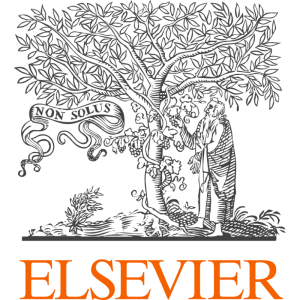
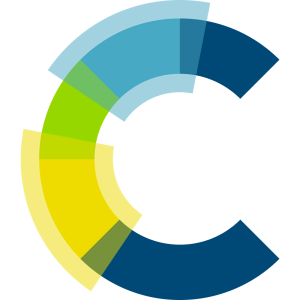
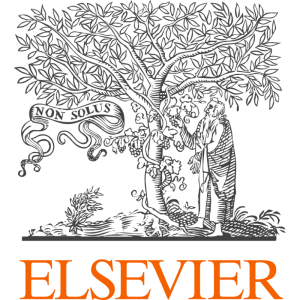
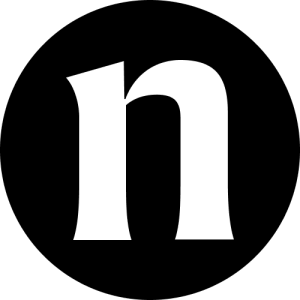
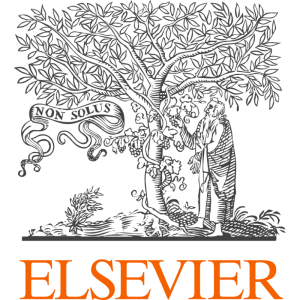
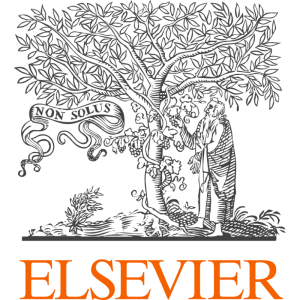
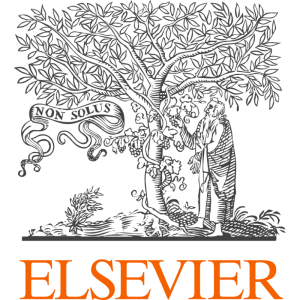
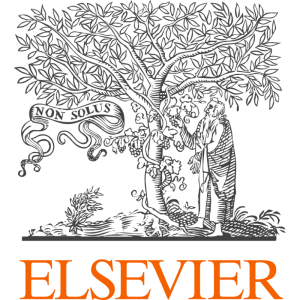
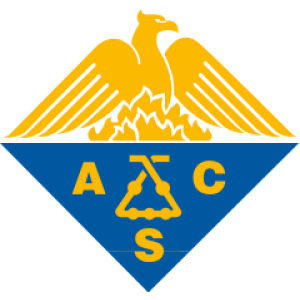
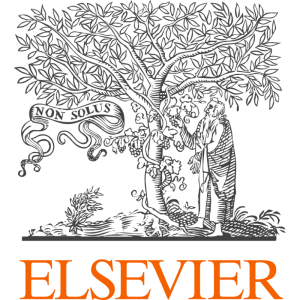
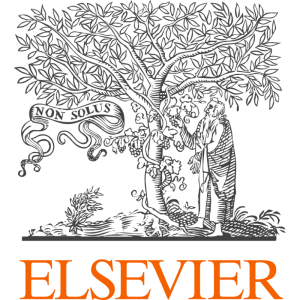
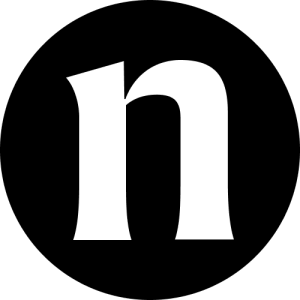
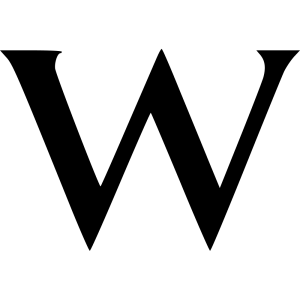
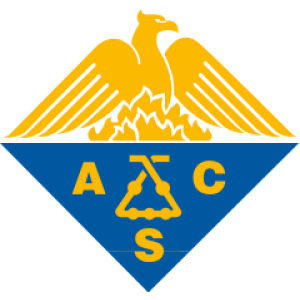
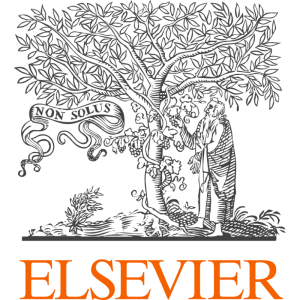
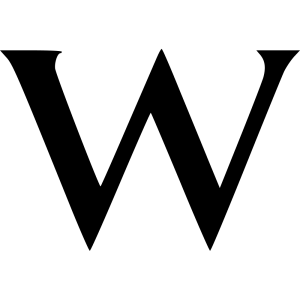
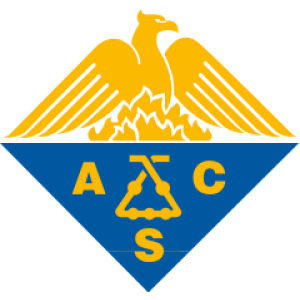
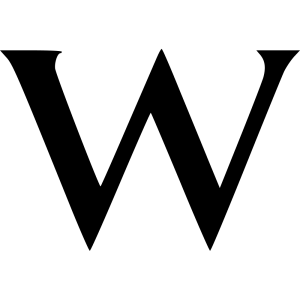
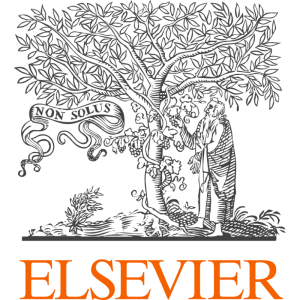
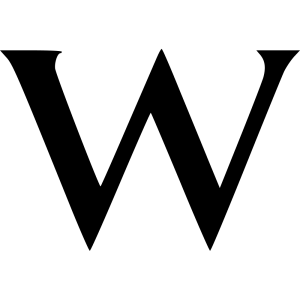
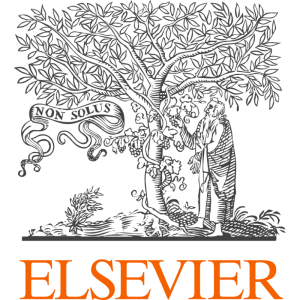
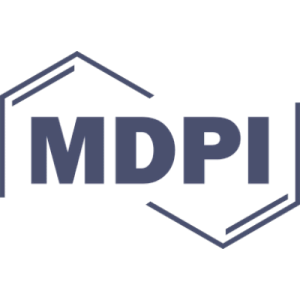
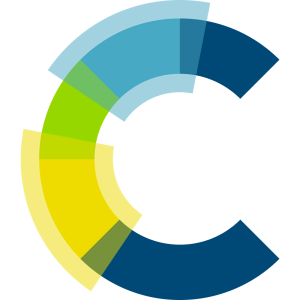
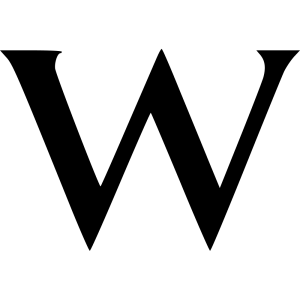
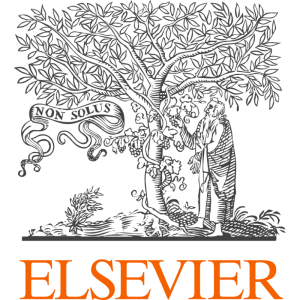
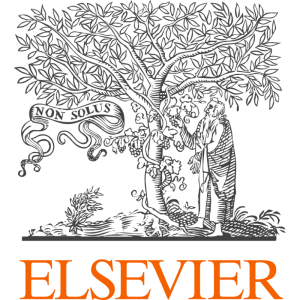
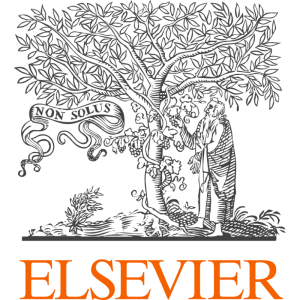
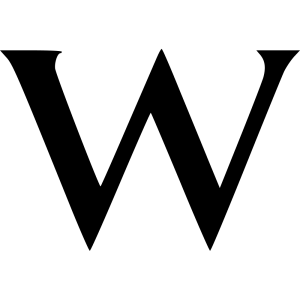
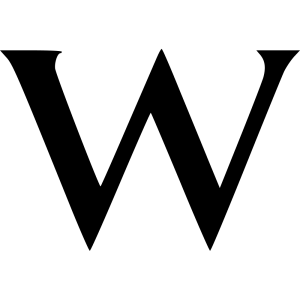
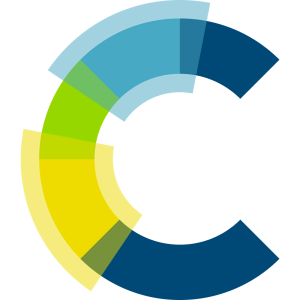
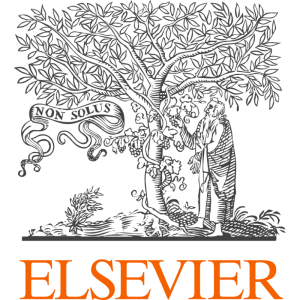
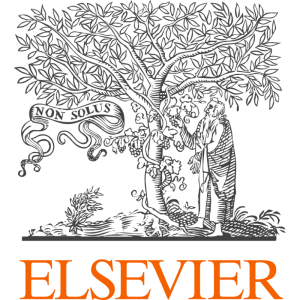
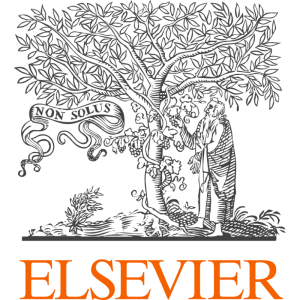
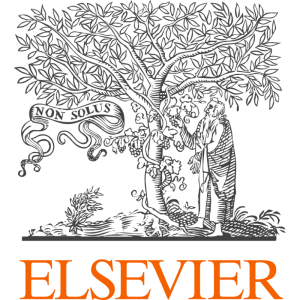
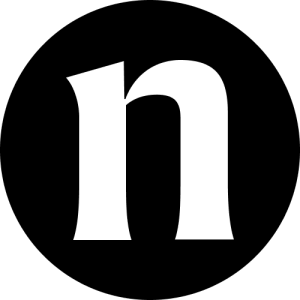
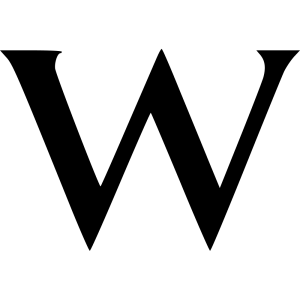
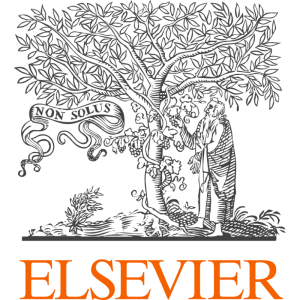
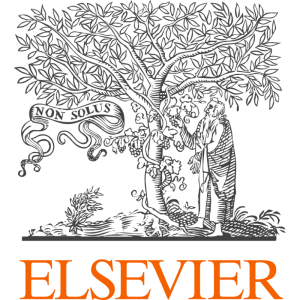
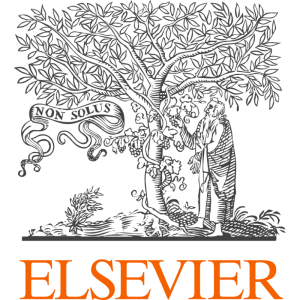
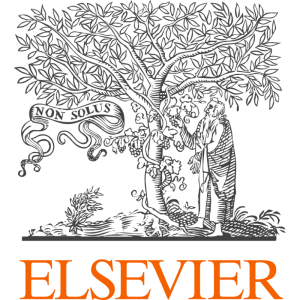
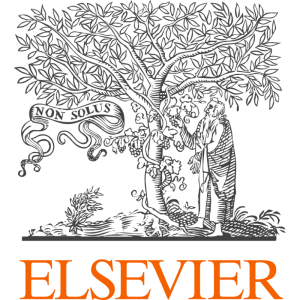
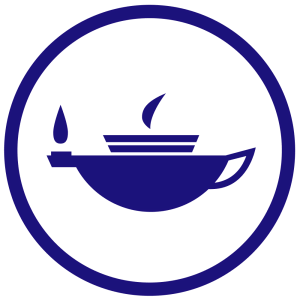
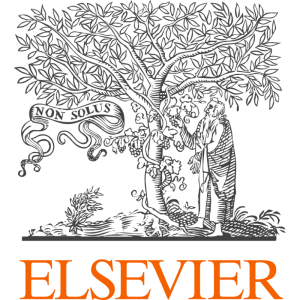
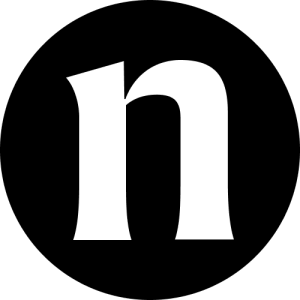
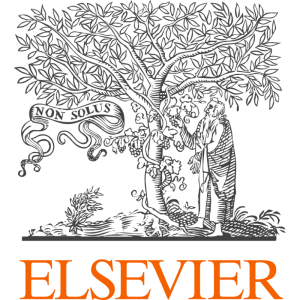
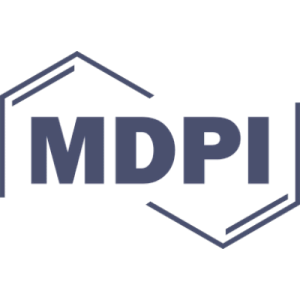
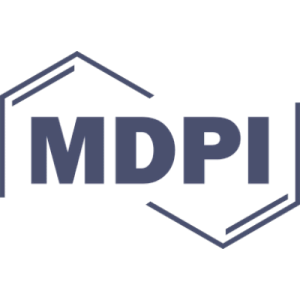
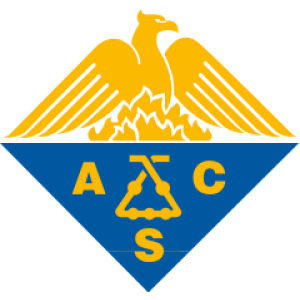
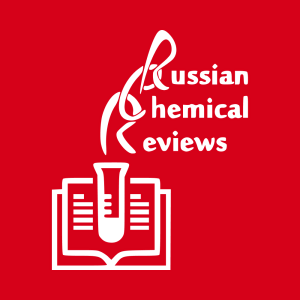
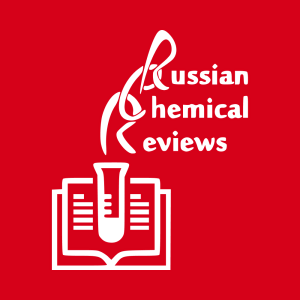
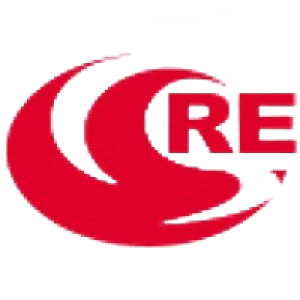
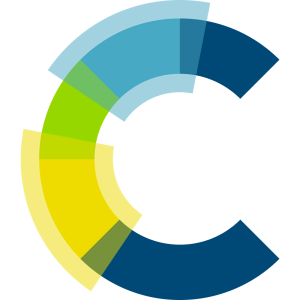
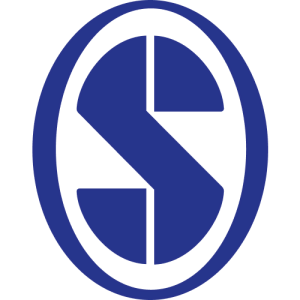
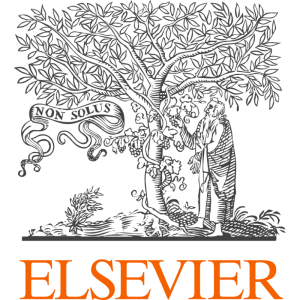
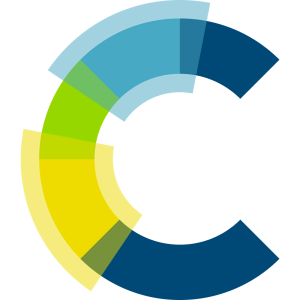
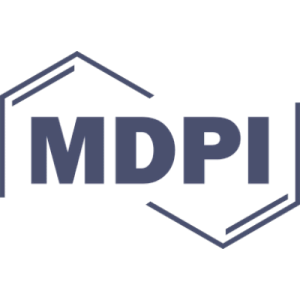
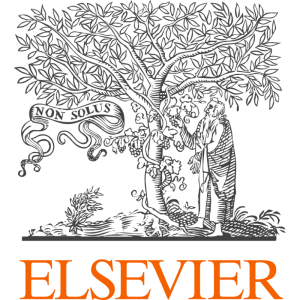
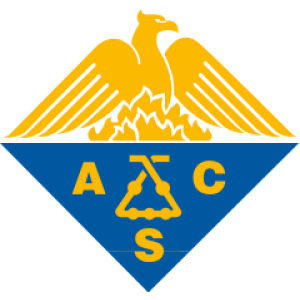
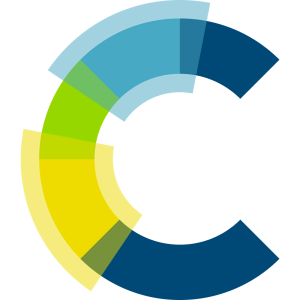
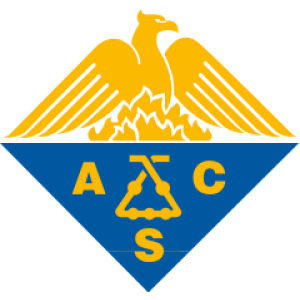
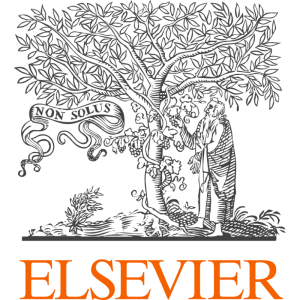
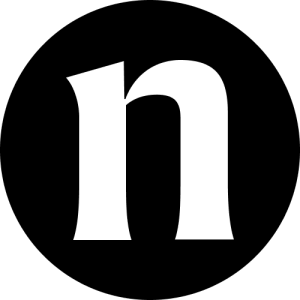
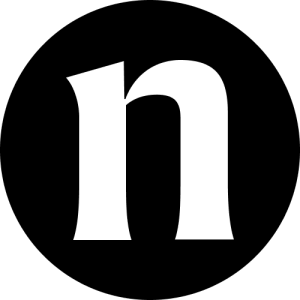
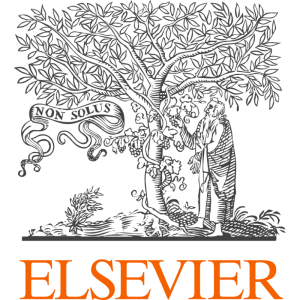
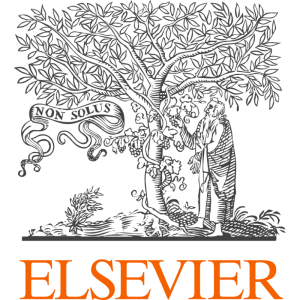
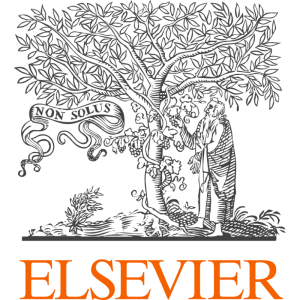
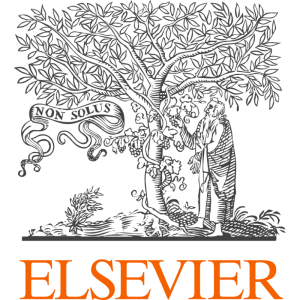
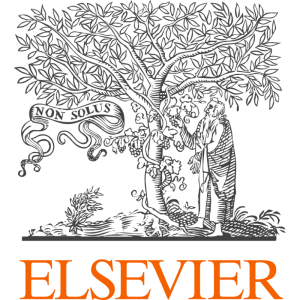
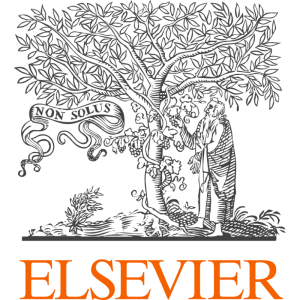
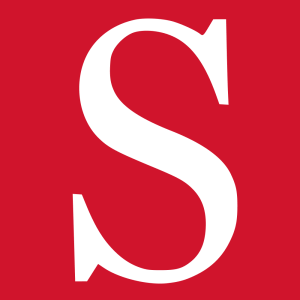
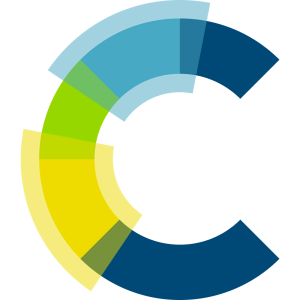
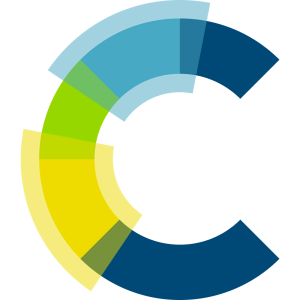
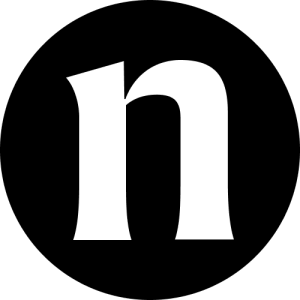
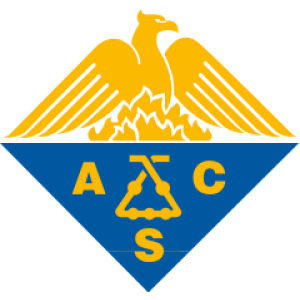
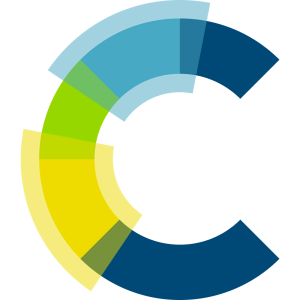
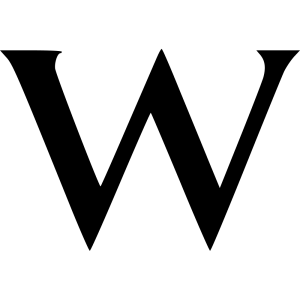
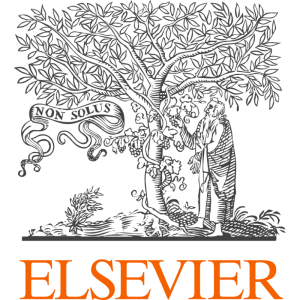
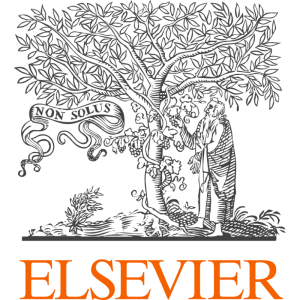
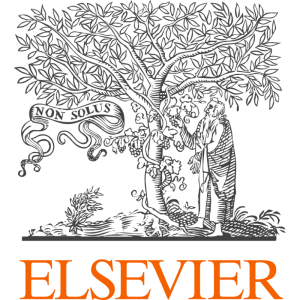
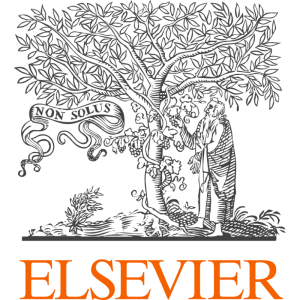
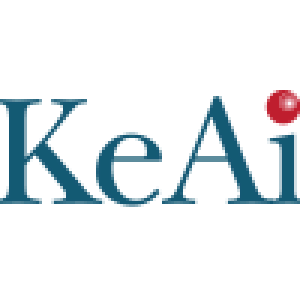
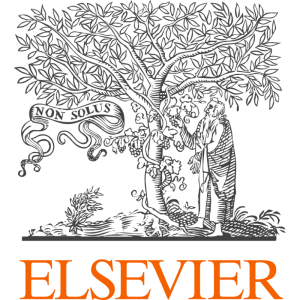
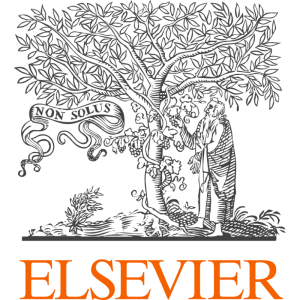
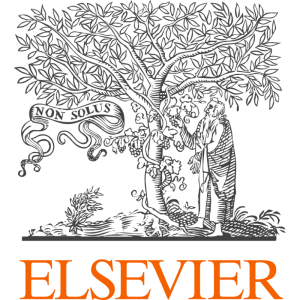
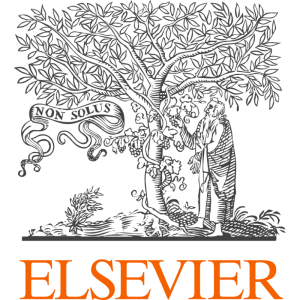
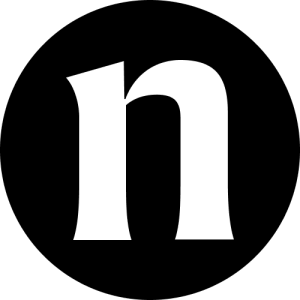
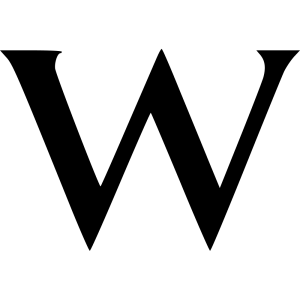
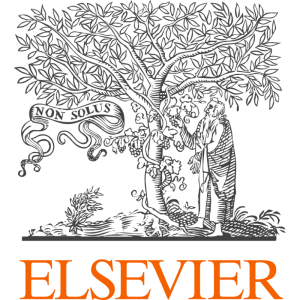
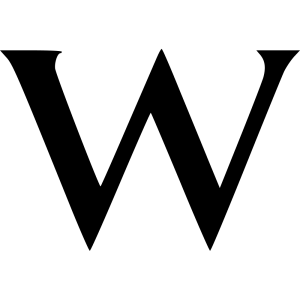
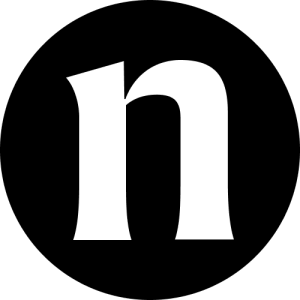
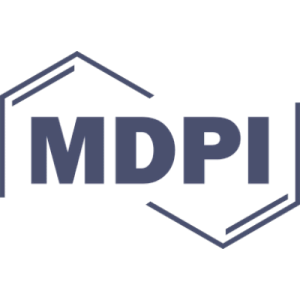
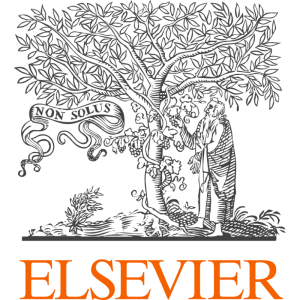
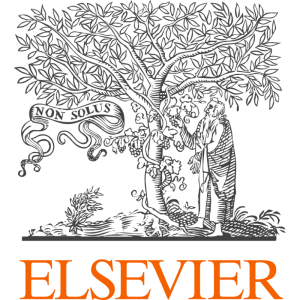
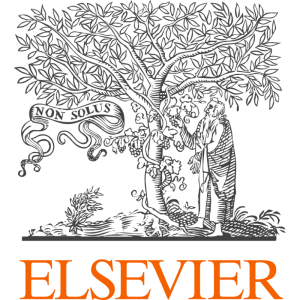
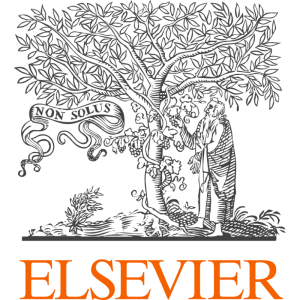
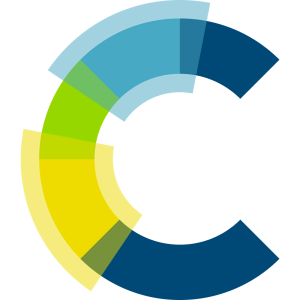
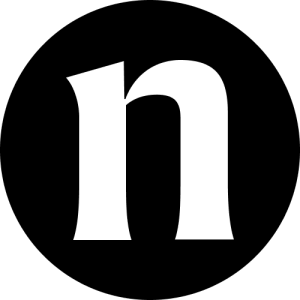
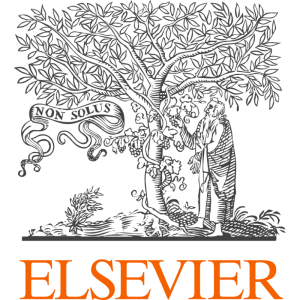
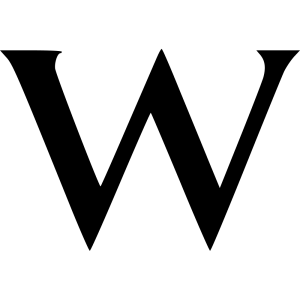
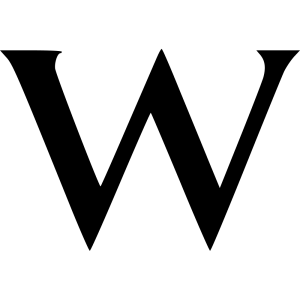
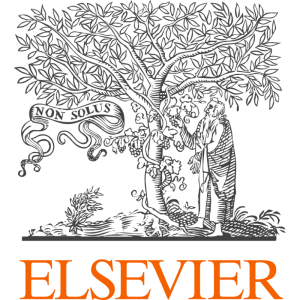
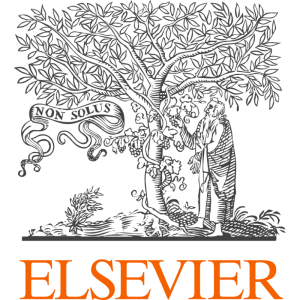
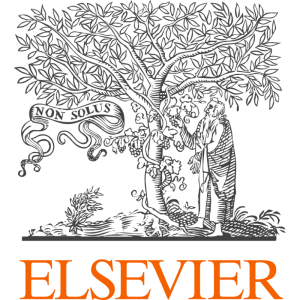
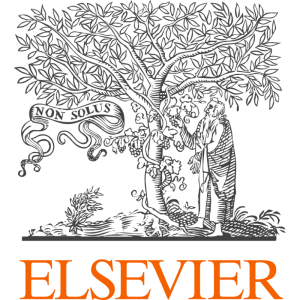
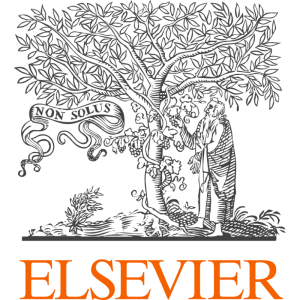
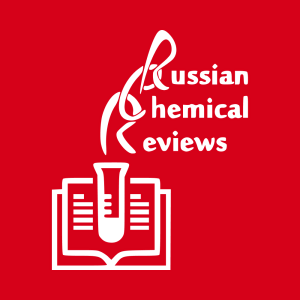
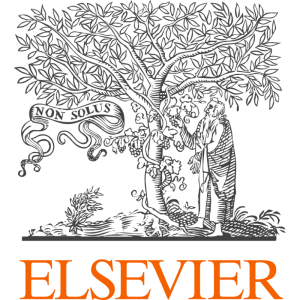
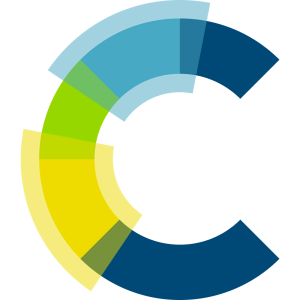
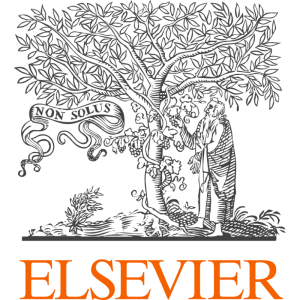
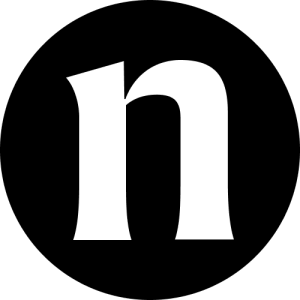
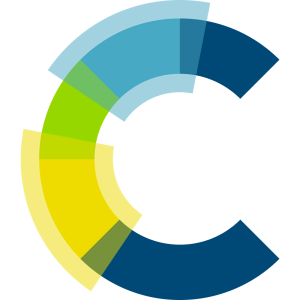
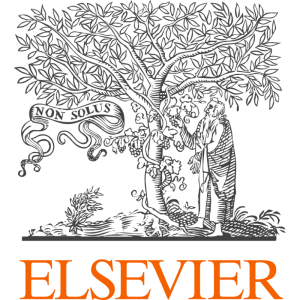
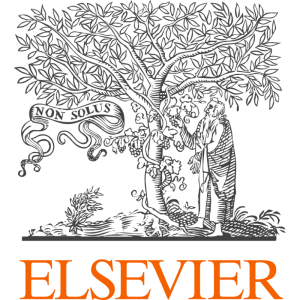
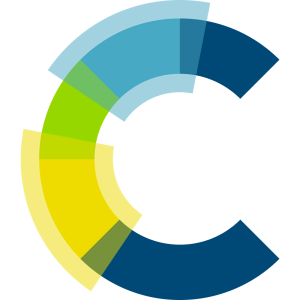
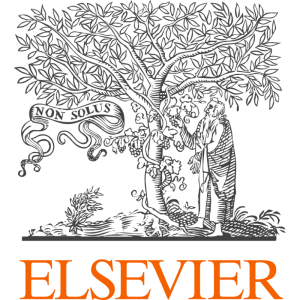
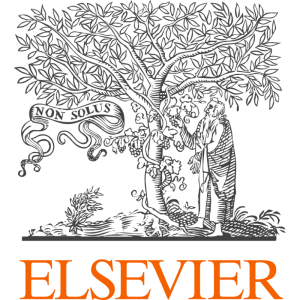
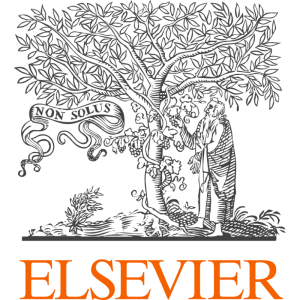
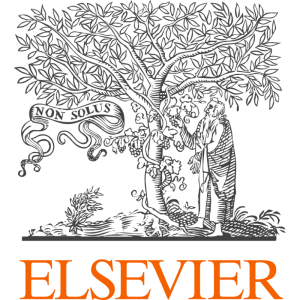
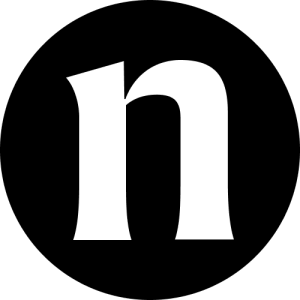
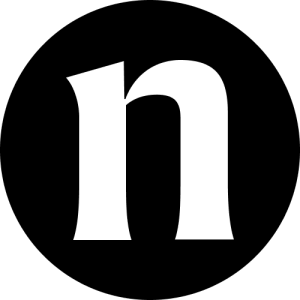
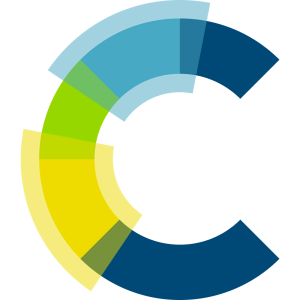
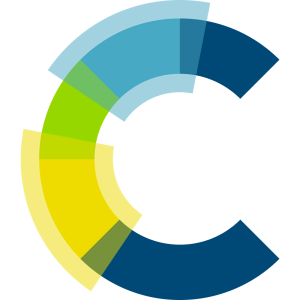
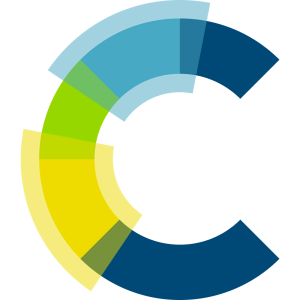
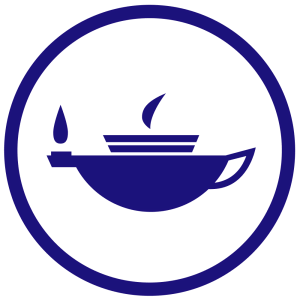
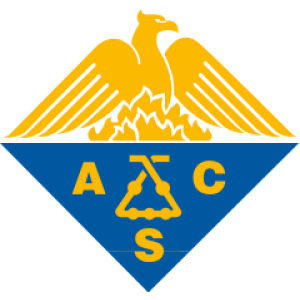
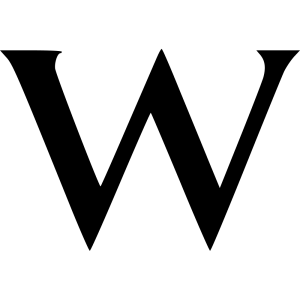
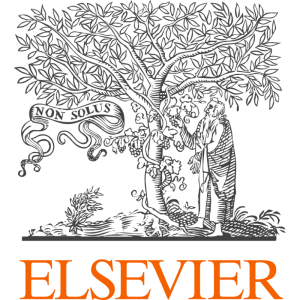
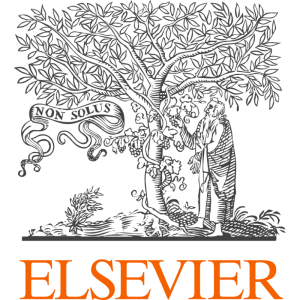
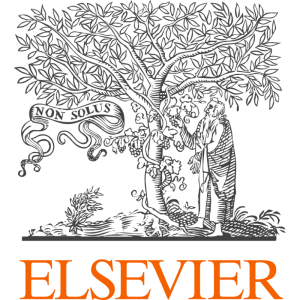
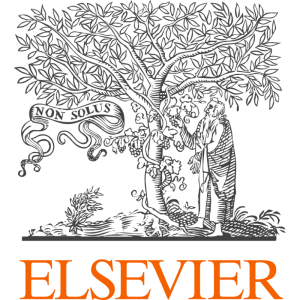
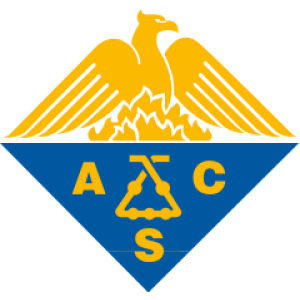
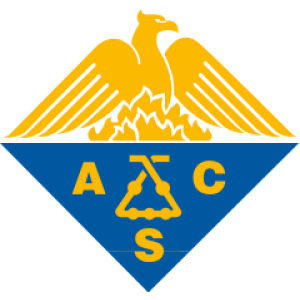
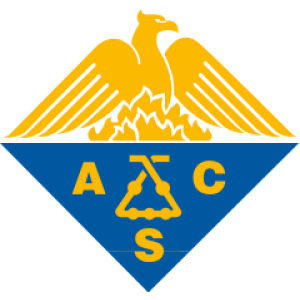
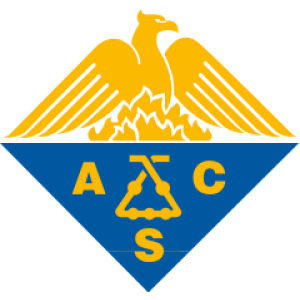
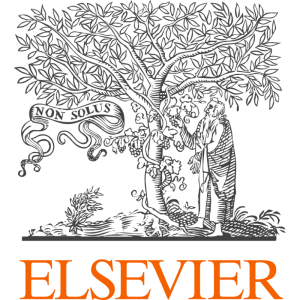
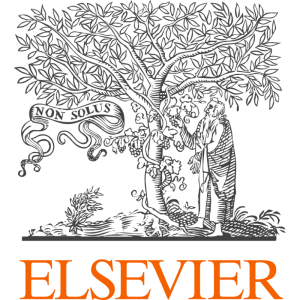
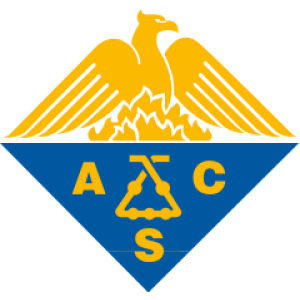
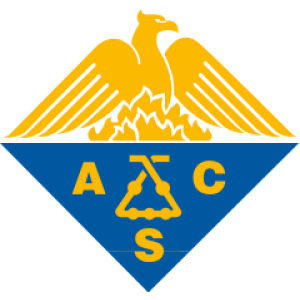
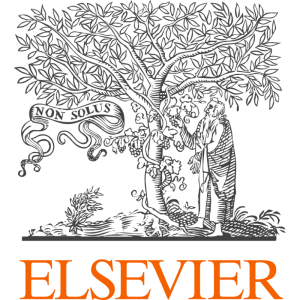
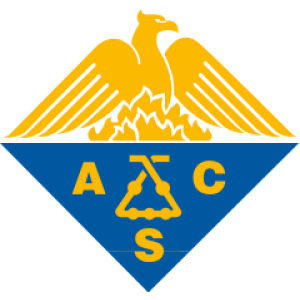
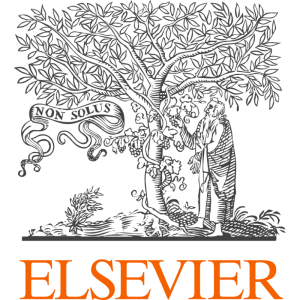
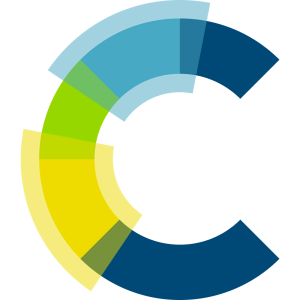
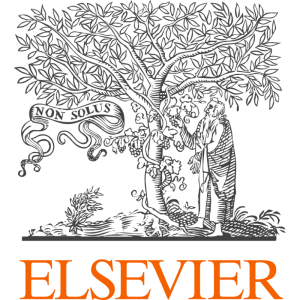
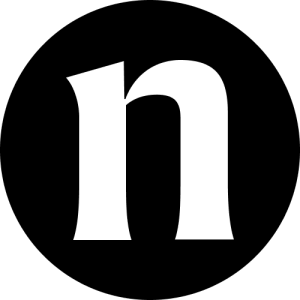
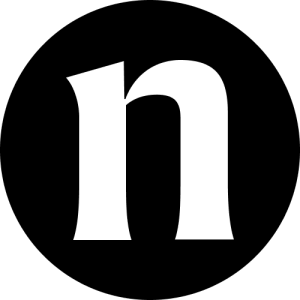
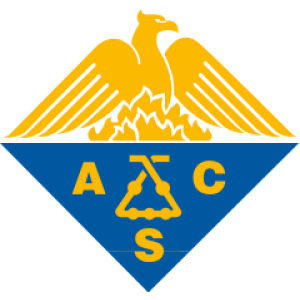
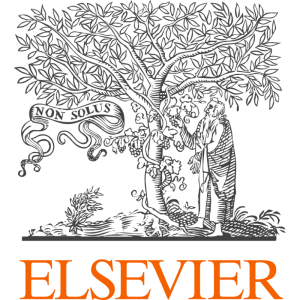
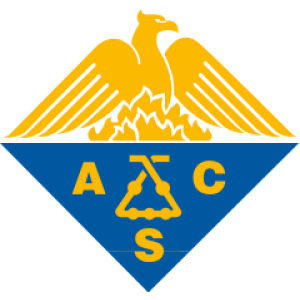
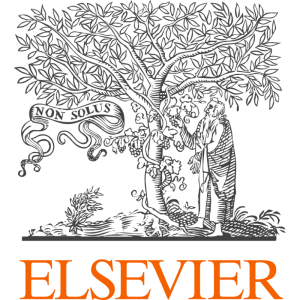
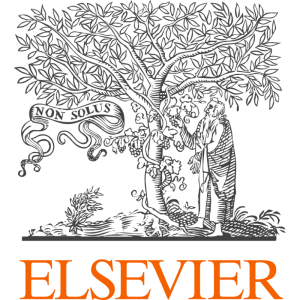
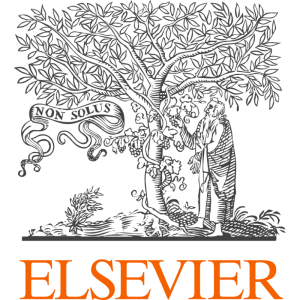
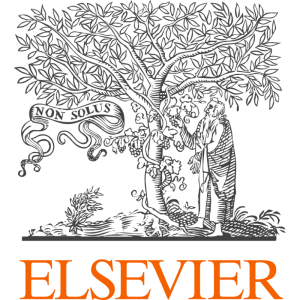
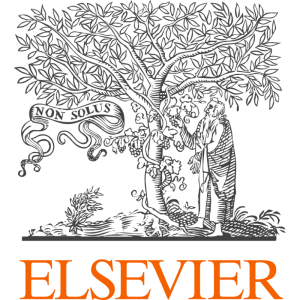
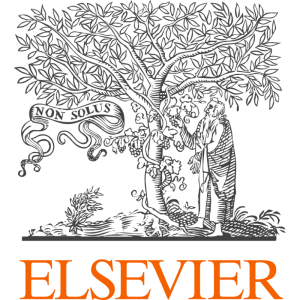
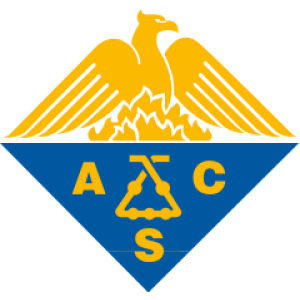
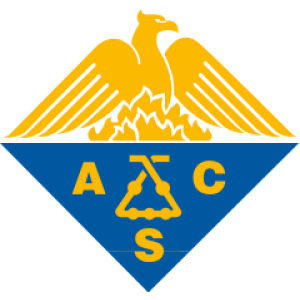
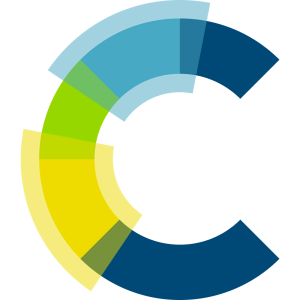
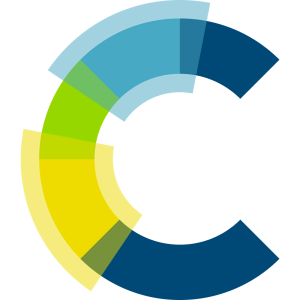
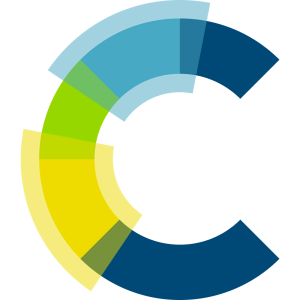
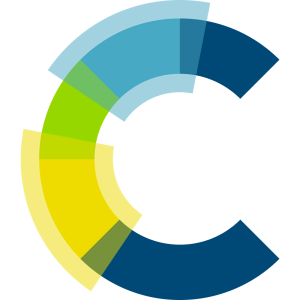
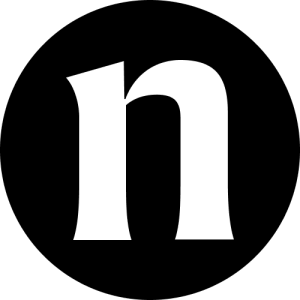
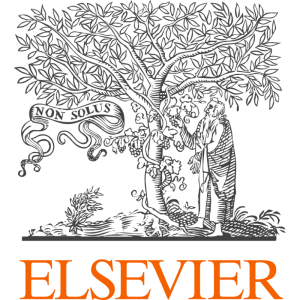
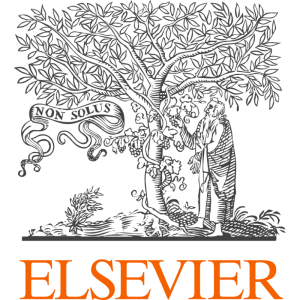
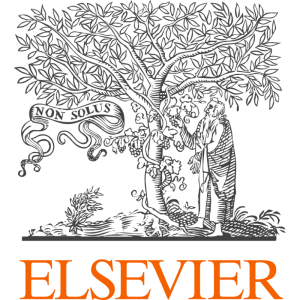
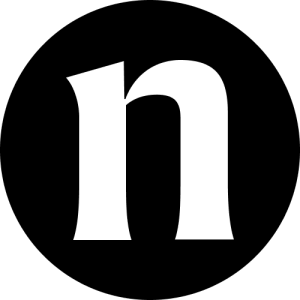
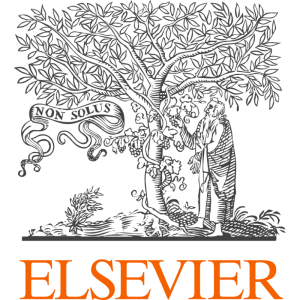
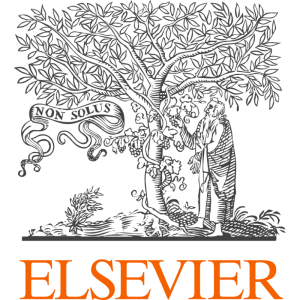
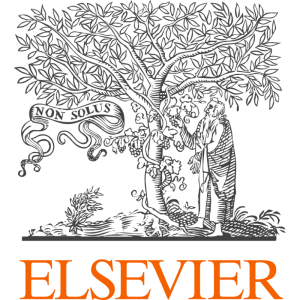
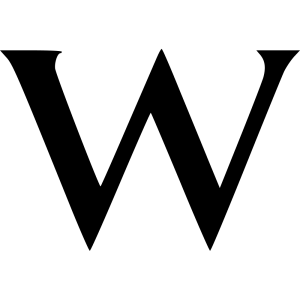
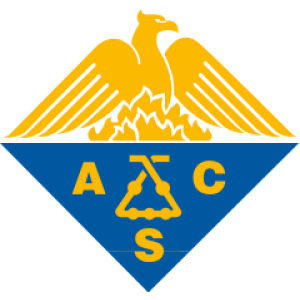
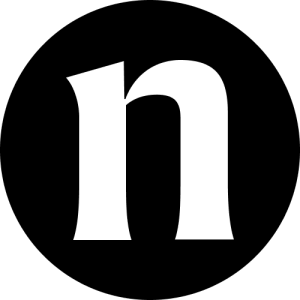
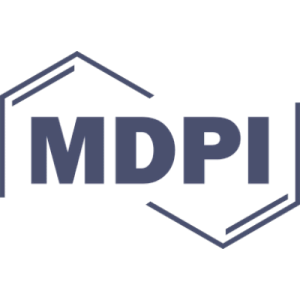
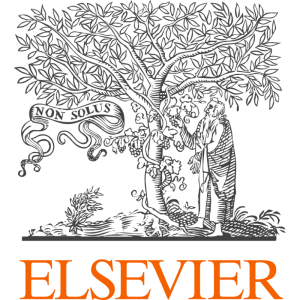
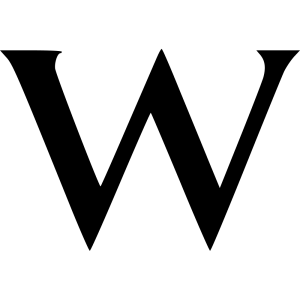
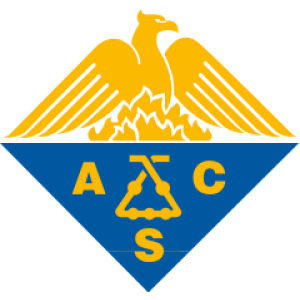
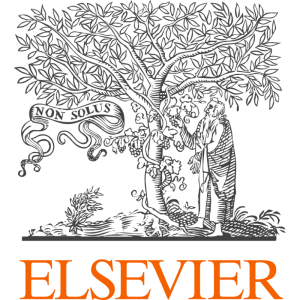
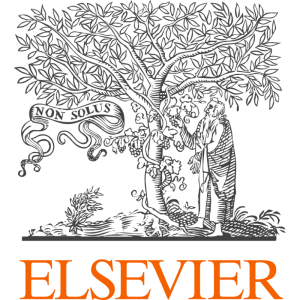
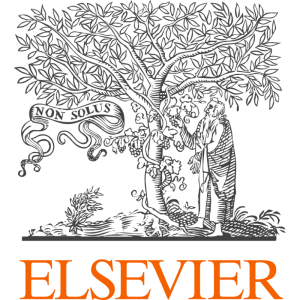
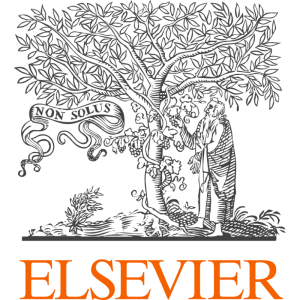
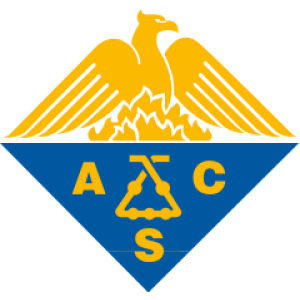
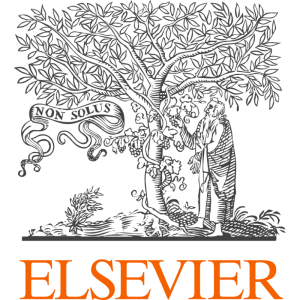
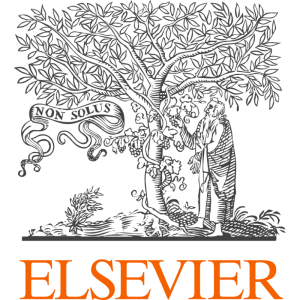
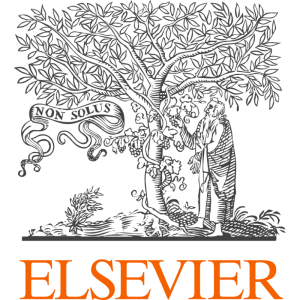
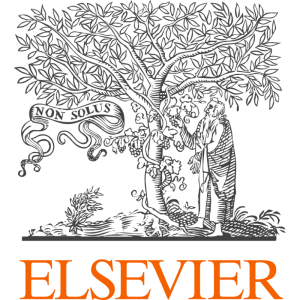
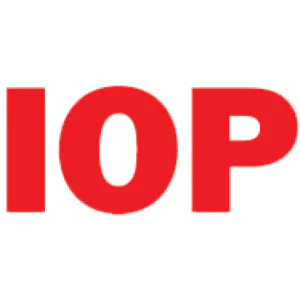
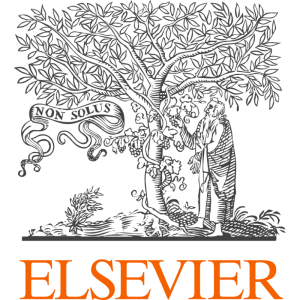
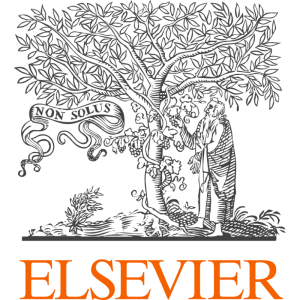
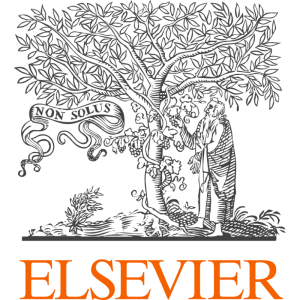
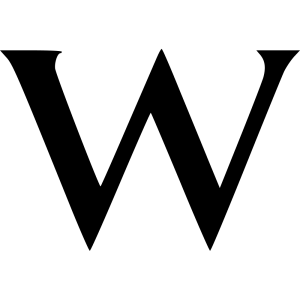
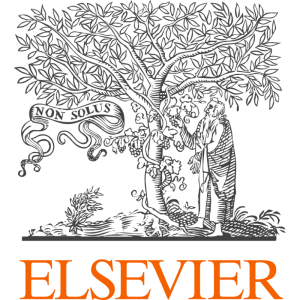
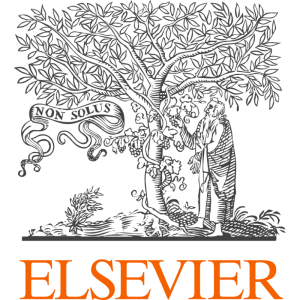
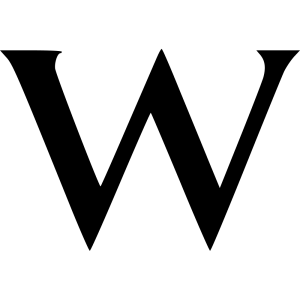
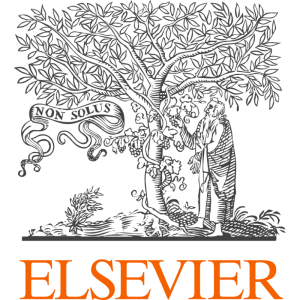
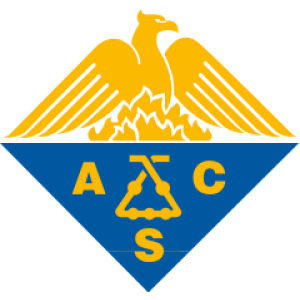
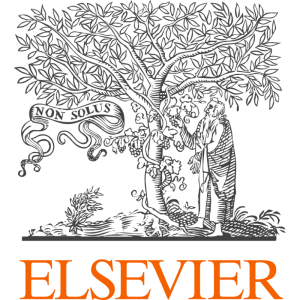
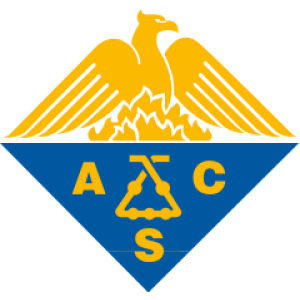
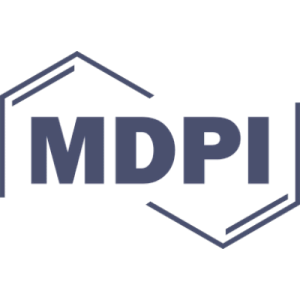
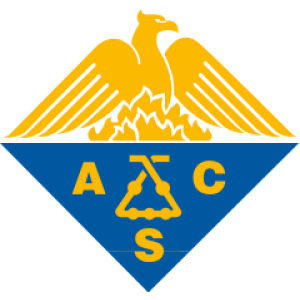
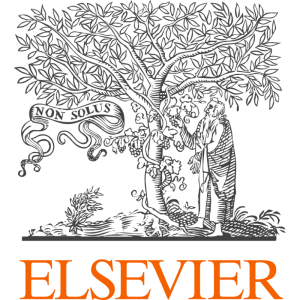
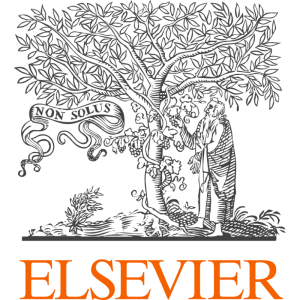
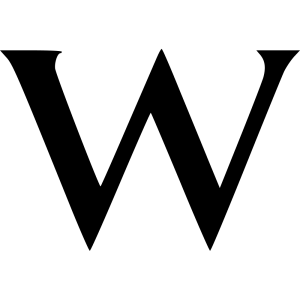
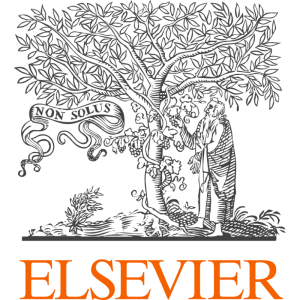
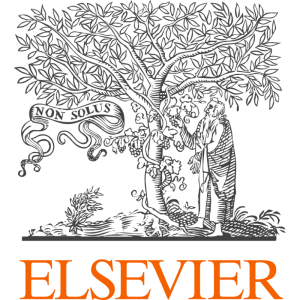
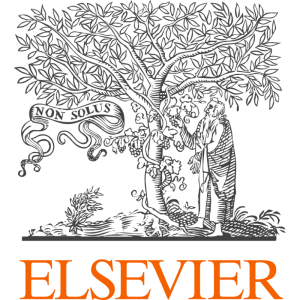
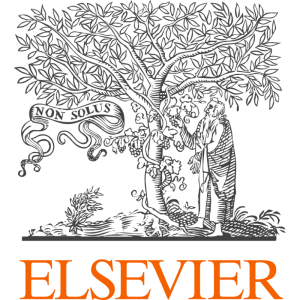
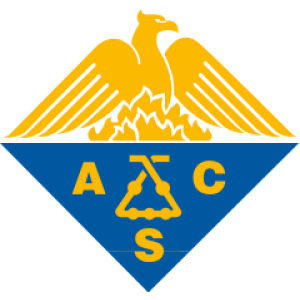
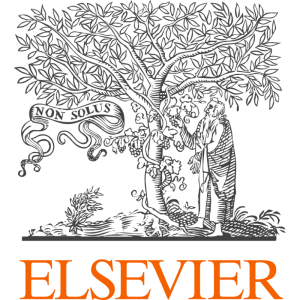
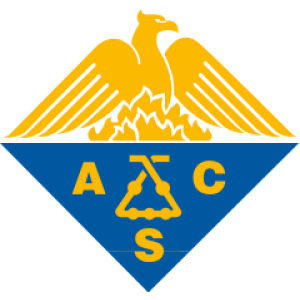
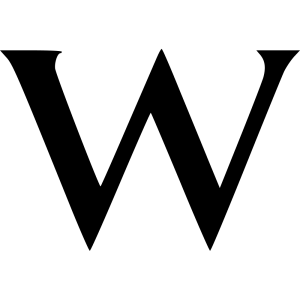
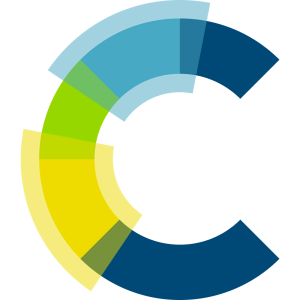
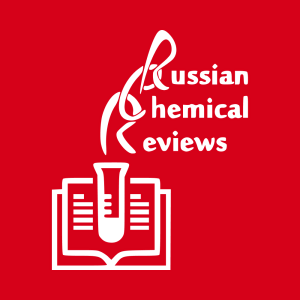