Keywords
Abstract
The COVID-19 epidemic demanded the rapid development of high-affinity molecules of different types aimed at a single target, the S-protein of SARS-CoV-2. The simultaneous development and testing of such molecules provide a unique opportunity to compare the features of biotechnological platforms for creating therapeutic proteins. This review considers classical antibodies, variable lymphocyte receptors, single-domain antibodies, and artificial scaffolds (DARPins, affibodies, VH), that are compared in terms of affinity, neutralizing activity, size and compatibility with different delivery methods. It can be concluded that all platforms used have produced high-affinity proteins that specifically bind to the coronavirus S-protein. The highest affinity of the targeting molecules with the virus protein was achieved by developing classical antibodies, nanobodies and by combining several binding modules into multivalent constructs with high avidity. Based on the results of in vivo experiments, it can be concluded that a high affinity of the therapeutic protein for the surface antigens of SARS-CoV-2 is a necessary but not sufficient condition for suppression of COVID-19 due to the peculiarities of the biology of this virus. The experience gained in the development of therapeutic agents against coronavirus will be useful for design of effective targeted drugs for the treatment of known and new viral infections.
The bibliography includes 126 references.
1. Introduction
A new coronavirus infection (COVID-19) was the first time reported in December 2019 and spread worldwide in a matter of months[1]. The world COVID-19 epidemic spread throughout the world, took at least 6 million lives[2], led to collapse of healthcare systems in many countries, and the fight against the spread of infection took a notable toll on the global economy. The main reason for the observed scale of the pandemic was the high efficiency of the spread of the SARS-COV-2 virus: it is airborne infection, with a basic reproduction number (R0) of 2.8 – 3.8 for early variants [3] and 5.08 for variant B.1.617.2 (‘Delta’)[4]. Later variants of the virus evolved to be even more transmissible: although variant B.1.1.529 (‘Omicron’) spread in a population of mostly recovered or vaccinated individuals, its effective reproduction number (Re) was 3.6, comparable to the replication number of the original virus in non-immune population. Basic R0 number for B.1.1.529 variant was estimated at 8.2[5]. Another reason for the rapid spread of the virus was the absence or low efficiency of the pre-existing adaptive immunity in the human population. In addition to SARS-COV-2, there are 6 other coronaviruses of the same family known to be pathogenic to humans: four of them cause seasonal acute respiratory infections and gastrointestinal tract infections[6], and two others have caused local epidemics with high mortality, namely SARS-CoV in 2003[7] and MERS-CoV in 2012[8].
A study of sera obtained in Singapore from healthy donors before the start of the COVID-19 pandemic showed that 55 to 96% of healthy people had antibodies to coronaviruses, primarily the seasonal cold viruses[9]. However, despite the high homology of conserved regions of the S-protein of related coronaviruses[10], antibodies to seasonal coronaviruses turned out to be ineffective against SARS-CoV-2[9].
Thus, most people apparently were not protected from COVID-19 by pre-existing adaptive immunity, and there was no ready-made specific pharmacological treatment.
The main factors of COVID-19 pathogenesis include:
1) reproduction of the virus in the respiratory tract, which can be accompanied by cough, fever, headache, muscle pain, and in severe cases of the disease can lead to shortness of breath and hypoxemia[11];
2) spread of the virus in the body causing damage to other organs, both direct and unrelated to virus replication (including pathologies of the intestines, kidneys, heart, liver, skin)[12];
3) systemic inflammation accompanied by the production of interleukin 1 (IL-1), 6 (IL-6), 8 (IL-8) and tumor necrosis factor (TNF) and an increase in the concentration of inflammatory markers in the blood (D-dimer and C-reactive protein);
4) coagulopathies associated with the formation of fibrin thrombi[11].
The fight against this disease was based on preventing the virus from entering cells, inhibiting virus replication in them, and addressing concomitant symptoms: hypoxemia, thrombosis, inflammation, and damage to non-respiratory organs[13]. Vaccination is considered the preferred method of preventing severe forms of COVID-19, but it is largely ineffective for therapeutic purposes when the virus infection occurs before vaccination. In addition, the emergence and spread of new variants of SARS-CoV-2 reduced the effectiveness of vaccination. Consequently, considerable material and intellectual resources of both individual research laboratories and pharmaceutical giants were devoted to the development of methods to suppress the infection that has already begun and to control the associated symptoms. Substances of various origins were considered to block the binding of the virus to cells: natural compounds[14], well-known pharmaceuticals[15], proteins[16], and new low-molecular weight ligands[17]. This review focuses on polypeptides and proteins that bind to viral surface proteins and have therapeutic potential. Such proteins are considered and compared according to their origin and properties: size, affinity, neutralizing ability, functional sites of molecules, the possibility of using them as part of recombinant constructs, and compatibility with various methods of delivery to the body (Table 1, Figure 1).
Most of the obtained protein molecules binding to the SARS-CoV-2 virion belong to antibodies. These proteins consist of 4 polypeptides; their antigen-binding surface is formed by two chains — the light and the heavy one (Figure 1). The variety of antibody specificities is provided by amino acid residues in hypervariable regions, located in the immunoglobulin domains of variable fragments[27]. Most often, antibodies of the G isotype (IgG), which have a maximum circulation time in the blood, were created for the treatment of COVID-19. Their constant part binds to the neonatal Fc receptor in the endosomes of endothelial cells, which ensures the return of antibodies to the blood plasma and prevents early destruction in lysosomes[28, 29]. An important property of antibodies, in addition to their high affinity, is the ability to recruit the immune cells, primarily natural killer (NK) cells, into interaction with infected cells[30, 31]. The diversity of antibodies in the body is created during the maturation of lymphocytes and somatic hypermutagenesis. Infection with a pathogen or immunization with an artificially obtained antigen ensures effective selection of high-affinity antibodies, that can then be further produced in vitro. A relative disadvantage of antibodies is their complexity: these glycoproteins with a quaternary structure, stabilized by disulfide bonds, can be produced only in mammalian cells[32].
Another important source of binding proteins is non-canonical antibodies. It is known that antibodies whose antigen-binding sites are localized within the same polypeptide chain have been discovered in camelids and sharks. This makes it possible to create shortened versions of single-domain antibodies (nanoantibodies, nanobodies, VHH), which can be produced in bacteria and used as binding modules in multifunctional fusion proteins. Besides, single-domain antibodies are relatively easily subjected to additional in vitro affinity enhancement using display technologies. Cyclostomes variable lymphocyte receptors (VLRs) are used similarly. These proteins of non-immunoglobulin nature are formed by an alpha-helical scaffold, which has variable regions that determine the specificity of the receptor.
Phage, ribosomal, and yeast displays used in the selection of high-affinity single-stranded and single-domain antibodies can also be used to create binding proteins from motifs of other origin. For this purpose, artificial scaffolds are used, which, like antibodies and VLRs, have a framework that allows replacing amino acid residues in several positions without losing the tertiary structure and selecting proteins with the highest affinity for the antigen. To create drugs specific to the SARS-CoV-2 virion, several types of such proteins were used: DARPins, variable sections of heavy chains of human immunoglobulin (VH), affibody, and miniproteins.
The process of developing and producing different types of proteins highly affinity to SARS-CoV-2 antigens is schematically shown in Figure 2.
Of all the protein types investigated, only monoclonal antibodies have been clinically used, which is more likely due to the ‘credit of trust’ that they had by 2020 than to their unique properties.
2. SARS-CoV-2 virion as a target of therapeutic proteins
The SARS-CoV-2 genome encodes 4 structural proteins of the virion, which could potentially become targets for therapeutic agents: the nucleocapsid protein N, associated with the viral RNA, and the membrane supercapsid proteins: spike protein S, envelope protein E, and membrane protein M[33]. However, proteins M and E are difficult to target, since they are transmembrane supercapsid proteins with epitopes minimally exposed on the surface of the virion: amino acid residues 1 – 18 out of 221 in the first case and 1 – 13 out of 75 in the second. N protein is located entirely within the virion and is inaccessible to antibodies and other binding proteins. The surface of the supercapsid membrane is largely shielded by the S-protein, which forms a homotrimer, which mediates the entry of the coronavirus into the infected cell. The protein part of this glycoprotein consists of 1273 amino acid residues (a.a.), forming the signal N-terminal peptide (1 – 13 a.a.), S1- (14 – 685 a.a.) and S2-domains (686 – 1273 a.a.). The S1 domain, in turn, consists of an N-terminal domain (NTD, 14 – 305 a.a.) and a receptor binding domain (RBD, 319 – 541 a.a.)[34]. The receptor-binding domain in the open conformation binds to angiotensin-converting enzyme 2 (angiotensin convertase, ACE2) on the surface of the human cell, and then the S-protein is cleaved by the membrane serine protease TMPRSS2[35]. An alternative to TMPRSS2 may be cathepsin L, which is also capable of cleaving the coronavirus S-protein in the endolysosome[36]. Cutting and conformational changes of the S-protein ensure the fusion of the membranes of the virus and the infected cell[37]. It is the S-protein, exposed on the surface of the virion and ensuring the penetration of the virus into the cell, that is the target of all therapeutic monoclonal antibodies and their analogues, summarized in Table 1.
All proteins listed in this table target the S-protein (the so-called ‘spike protein’) of the SARS-CoV-2 virion in order to prevent the virus from entering cells at the start of infection or at the time of virus spread in the body. Thus, the key characteristics of therapeutic proteins are their affinity for the S-proteins of different virus variants and their neutralizing ability in vitro. In addition, antibodies that attach to viral proteins on the surface of infected cells can activate NK cells through the constant moiety[38]. This ability is unique to antibodies and makes an additional contribution to the infection inhibition.
To date, at least three mechanisms of action of antibodies that prevent the virus from entering cells are known:
1) stabilization of the S-protein in a closed conformation,
2) blocking the binding of S-protein to angiotensin-converting enzyme[39], and
3) stabilization of the S-protein in a conformation that promotes its cleavage and separation of the S1 domain[40].
Antibodies binding to the N-terminal S1 domain (NTD) and not competing for the binding of RBD to angiotensin convertase also have neutralizing activity. Considering virus neutralization and epitope accessibility, proteins targeting the epitopes of the S1 domain of the spike protein, especially its receptor-binding domain, are of the greatest interest. Nevertheless, from the standpoint of the versatility of the drug, antibodies targeting the S2 domain may be promising. This region of the S-protein is more conservative and has high homology both in different strains of SARS-CoV-2 and in different coronaviruses[10, 41].
Testing SARS-CoV-2-specific proteins for neutralizing activity was the first step in the sequence of preclinical and clinical studies, schematically presented in Figure 3.
3. Platforms for the development of targeted proteins specific to SARS-CoV-2
3.1 Monoclonal antibodies
Antibodies are natural protective molecules, that are able to bind specifically to the target antigen, including blocking its interactions with other molecules, and also have effector functions mediated by the constant Fc-fragment. Most of the neutralizing antibodies bind to the S-protein of the coronavirus and prevent its interaction with the angiotensin convertase, receptor protein on the host cell surface[39]. The question of how many antibodies to SARS-CoV-2 antigens arising in patients after vaccination or during infection is necessary and sufficient for COVID-19 treatment needs to be specifically investigated. Thus, in a sample of Moscow patients in 2020, it was shown that part of the patients that had recovered after COVID-19 had no SARS-CoV-2 neutralizing antibodies in their blood[42] (from 10 to 20% of patients, depending on the method of analysis), and this did not prevent the recovering process. A similar study[43], conducted in London, found that virus-specific antibodies 2 months after infection were absent in a proportion of patients that can be estimated to be between 2 and 8.5%. Furthermore, in the case of direct intercellular transmission of the virus from infected to uninfected neighboring cell, the concentration of neutralizing antibodies should be an order of magnitude higher than that effective in infection transmission by circulating viral particles[44]. For this reason, despite widespread attempts to use polyclonal antibody sera taken from survivors of the pandemic (convalescents) during the initial period of the pandemic[45], subsequently, only monoclonal antibodies were preferred in the treatment of COVID-19. The screening strategy for such antibodies was straightforward in most cases: selection of memory B cells of COVID-19 patient for binding to S-protein, sequencing of loci encoding V-domains, cloning of recombinant antibodies, in vitro tests on neutralizing activity, and in vivo tests on animal models. This strategy was first described in April 2020 by a team of Chinese authors[46] for the CB6 antibody, which was later used in the treatment of COVID-19 as etesevimab.
The first neutralizing SARS-CoV-2 monoclonal antibody approved for medical use was bamlanivimab. Initially, this drug was found during the antibody screening of a Chinese COVID-19 patient and named LY-CoV555. The screening procedure was as follows: 20 days after the diagnosis of COVID-19 symptoms, 2238 single B cells producing antibodies binding to the trimerized spike protein were taken from the patient, and heavy and light chain loci were sequenced for 440 cells[47]. Bioinformatic analysis selected 187 candidate antibodies, and then 175 were cloned into producing cell line. The ability to bind to wild-type S-protein in vitro was confirmed for 77% of antibodies, and 14% of antibodies could competitively inhibit S-protein binding to ACE2. IgG1 bamlanivimab was selected from among them due to the lowest concentration (about 100 μg L–1), effective in blocking infection of Vero cells by reporter viral particles in vitro. The affinty to the S-protein (dissociation constant of the antigen – antibody complex) for bamlanivimab was ~10–9 mol L–1[48].
The first clinical trials showed that when using bamlanivimab at a dosage of 7000 mg per person, there was no significant effect on the course of COVID-19. Although in the group of patients undergoing treatment, the proportion of severe disease was higher than in the group receiving placebo, and one fatal outcome was also recorded[49]. At the same time, the introduction of such an amount of recombinant protein into the bloodstream required an intravenous infusion lasting 1 hour. Together with bamlanivimab, another monoclonal antibody, etesevimab (alternative names CB6, LY3832479, LY-CoV016), and the combined effect had an effect on reducing viral load, unlike bamlanivimab monotherapy[50]. The half-maximal inhibition concentration (IC50) for the neutralizing activity of bamlanivimab was 31 μg L–1, and for etesevimab it was 189 μg L–1[51]. Despite conflicting data on therapeutic efficacy, bamlanivimab has been approved for use by the U.S. Food and Drug Administration (FDA) both in combination with etesevimab and as monotherapy[52].
The regdanvimab antibody (CT-P59) was obtained as a result of screening a library of single-stranded V-domains of antibodies by phage display from a patient from South Korea[53]. To create a library, peripheral blood mononuclear leukocytes were taken from the patient and RNA was isolated. Further, cDNA was obtained, VH– and VL– domain encoding sequences were amplified by PCR, and then cloned together into a phagmid in scFv format. The resulting phage library was repeatedly enriched with magnetic beads coated with recombinant RBD domain of the SARS-CoV-2 S-protein. The best clones were selected using solid-phase enzyme immunoassay (ELISA), for further work they were cloned from the scFv format into the format of full-size human antibodies and expressed in the Chinese hamster ovarian cells (CHO). Full-size antibodies were analyzed in vitro on Vero cells, determining their neutralizing activity, and affinity to the RBD domain, determined by surface plasmon resonance (SPR). IC50 for neutralizing activity was 5.7 ng ml–1, the dissociation constant for the S-protein was 2.7 × 10–11 mol L–1. Further, the therapeutic activity of regdanvimab was determined in preclinical studies on ferrets, golden Syrian hamsters and rhesus macaques[53]. In clinical trials, regdanvimab was used in dosages ranging from 20 to 80 mg per 1 kg of patient weight, however, a small number of participants (3 people in the control group and 15 people in three groups receiving the antibody) prevented reliable conclusions about its efficacy[54].
Another antibody sotrovimab (VIR-7831) was developed based on the S309 antibody, obtained from the cells of a SARS patient in 2003[55]. Work on its creation began with screening of 25 antibodies to the SARS-CoV virus S-protein for cross-reactive binding to the surface of CHO cells expressing the SARS-CoV-2 virus S-protein. For 8 antibodies that showed binding to the new S-protein, in vitro tests were performed to determine their neutralizing activity on Vero cells. The S309 antibody demonstrated the best results: the IC50 value was 79 μg L–1, the dissociation constant of the S-protein complex is 1.5 × 10–11 mol L–1. Sotrovimab was created by making changes in the sequence of the Fc-fragment of the S309 antibody, improving binding to the neonatal Fc-receptor and increasing the circulation time of the antibody in the bloodstream[56]. Clinical trials have shown its effectiveness: when taking this drug, the risks of hospitalization of patients with COVID-19 decreased to 1%[57].
Kazirivimab (REGN10933) and imdevimab (REGN10987), another two monoclonal antibodies, simultaneously used as a cocktail of REGN-CoV2 antibodies, were obtained from co-screening of immunized VelocImmune transgenic mice, with fully humanized immunoglobulin loci, and B cells from a COVID-19 patient[58]. Lymphocytes were stained with fluorescent labeled recombinant RBD, and specific B cells were sorted by fluorescence-mediated cell sorting. Recombinant antibodies were cloned and expressed in the producing CHO cell line. Antibodies were selected based on the analysis of neutralizing activity in vitro using the S-protein decorated VSV virus and Vero cells. Coding sequences were sequenced for 253 antibodies that showed neutralizing activity, and epitopes were mapped for 9 of the most effective ones. According to the overlap of epitopes, 4 groups were identified. REGN10987 antibodies from the first group and REGN10933 from the second group were selected to create a therapeutic cocktail because their binding to the RBD-domain was independent of each other. Values IC50 for the neutralizing activity of imdevimab and casirivimab were 2.3 × 10–10 and 6.9 × 10–11 mol L–1 (≈35 and 11 μg L–1), the dissociation constants of complexes with S-protein were 4.3 × 10–11 and 4.2 × 10–11 mol L–1, respectively.
Clinical trials on hospitalized patients receiving 4 g of imdevimab and casirivimab by intravenous infusion showed that such treatment reduced mortality from COVID-19 in British hospitals from 30 to 24%[59]. However, the therapeutic effect of using the casirivimab/imdevimab composition was moderate. Given the limited availability of the drug, since September 2021, a cocktail of REGN-CoV2 antibodies has been recommended for patients with severe disease if they are seronegative and for patients at high risk of hospitalization[60, 61].
Tixagevimab (AZD8895, COV2-2196) and silgavimab (AZD1061, COV2-2130) were obtained by analyzing the antibodies of the first four Americans infected with this coronavirus in China in early 2020. B cells were stained with the RBD domain of the S-protein fused with the Fc-fragment (Fc is a crystallizing fragment of immunoglobulin) of mouse antibodies, and was isolated by fluorescence-mediated cell sorting. Then isolated memory B cells were stimulated by BAFF, IL-21 and CD40L and secreted antibodies were tested for binding to recombinant S-protein domains and for the ability to neutralize in vitro the Wuhan variant of SARS-CoV-2 supplemented by the luciferase gene. Slightly more than half (178) of the 389 antibodies obtained, recognized RBD-domain, including 67 antibodies that were neutralizing[62]. Antibody coding loci were sequenced, among them 321 unique sequences were identified. For 49 antibodies, the neutralizing activity was confirmed in vitro using the S-protein-decorated VSV virus. Two antibodies with the best antigen binding rates, on the basis of which tixagevimab and silgavimab were further obtained, showed IC50 values for neutralizing activity in relation to the initial SARS-CoV-2 variant of 15 and 107 μg L–1, respectively; they did not compete for the epitope, which allowed them to be used together[63]. Curiously, the IC50 values for neutralizing activity against pseudovirus for the same antibodies were 0.7 and 1.5 μg L–1, which may indicate shortcomings of the pseudovirus system for determining neutralizing activity. For the S-protein bound to tixagevimab and silgavimab, a three-dimensional structure of the complex was obtained, which confirmed its complementary blocking by antibodies[64].
Clinical trials to determine the therapeutic effect of the tixagevimab/silgavimab cocktail at a dosage of 300 mg of each antibody intravenously showed a decrease in mortality in patients hospitalized with COVID-19 from 12 to 9%[65]. Prophylactic intramuscular administration of the same cocktail at a similar dosage in some cases looked encouraging: 0.2 and 4.4% of COVID-19 patients vs. 1.0 and 8.9% in the placebo group (see Refs [66, 67], respectively); at that time as in another study [68] there was no significant reduction in morbidity: 3.1% of cases vs. 4.6% in the placebo group.
Chronologically the last therapeutic antibody to S-protein was bebtelovimab (LYCoV1404), obtained as a result of screening of lymphocytes of a patient who had COVID-19[51]. At the first stage, using in vitro tests for binding of B cells to recombinant S-protein, as well as with S-protein-coated beads and cells expressing S-protein, it was possible to select 1062 antibody synthesizing cells, then the library of V-domain sequences was sequenced and isolated for 290 paired combinations of VH – VL domains. Based on them, after bioinformatic analysis 69 antibodies were selected to obtain recombinant antibodies, for which the affinity to the S-protein was determined by surface plasmon resonance and epitopes were mapped. As a result, bebtelovimab was selected from among others, since it bound the same S-protein epitope as the previously obtained antibodies sotronivimab and imdevimab. The dissociation constant of bebtelovimab and S-protein was 7.5 × 10–11 mol L–1, the IC50 value for neutralizing activity was 9 μg L–1[51].
Changes in the sequence of the S-protein during evolution led to an increase in its affinity for ACE2 and a decrease in its affinity for therapeutic antibodies. For example, N501Y amino acid replacement changed the affinity for ACE2 by an order of magnitude from 5.8 × 10–9 to 5.7 × 10–10 mol L–1[48]. Coronavirus variant B.1.1.529, better known as the omicron variant[69], discovered in November 2021, turned out to be insensitive to licensed therapeutic monoclonal antibodies. The spread of this strain and its new variants has led to a further revision of the clinical recommendations of the World Health Organization (WHO). For example, in March 2022 WHO recommended the use of the combination casirivimab/imdevimab only if it was possible to quickly determine the genotype of the virus and its strain was sensitive to these drugs[70, 71].
In January 2022, the US FDA retrieved the use permits issued to the antibody compositions bamlanivimab/etesevimab and kazirivimab/imdevimab, citing their ineffectiveness; however, after that, even more than 150 thousand doses of these monoclonal antibodies were used in the USA[72].
It should be noted that some neutralizing antibodies isolated from patients did not reached the clinical use [73] but nevertheless showed high neutralizing activity in vitro against evolutionarily later variants of the virus compared to the original SARS-CoV-2. Thus, for the iB20 antibody, the neutralizing activity (IC50) for the Wuhan variant was 110 μg L–1, and for the variant ‘omicron’ it was 18 μg L–1, although the KD predicted the opposite relation: 5.7 × 10–10 mol L–1 for the initial version and 4.5 × 10–9 mol L–1 for Omicron[74].
To date, approvals have been withdrawn for all antibody-based drugs specific to the SARS-CoV-2 S-protein.
3.2. High affinity molecules of camelids and cyclostomes
In addition to classical mouse and human monoclonal antibodies, single-domain antibodies of camelids (camels, llamas, and alpacas) specific to SARS-CoV-2 proteins were generated. Such molecules are attractive because their entire antigen-binding site is located in a single polypeptide sequence as part of a variable heavy chain domain called VHH. This facilitates the genetic engineering of antibodies, including the production of shortened forms (nanoantibodies) and their assembly into bi- and multivalent designs. Nanoantibodies (nanobodies) can be produced in bacteria, and due to their chemical properties, these compounds can be used in the form of aerosols against respiratory viral infections[75, 76]. In addition, differences in the structure of molecules make it possible to obtain variants that recognize epitopes that are inaccessible to mouse and human antibodies. Thus, the antibodies of representatives of camelids have a greater flexibility of the paratope loops and more extensive hypervariable areas[77].
The relative disadvantage of nanoantibodies is the difficulty of working with the large animals that are expensive to maintain and difficult to immunize. However, in vitro selection methods make it possible to select nanoantibodies from synthetic libraries using a phage or yeast display without prior immunization of animals: about two thirds of the obtained nanoantibodies specific to the coronavirus S-protein were selected in this way[39].
In another method of selection of nanoantibodies was proposed[78], it does not require immunization of camelids. The authors obtained S-protein-specific nanobodies using transgenic mice. The researchers inserted a cassette containing 18 alpaca VHH sequences into the mouse immunoglobulin heavy chain locus, 7 dromedary camel VHH sequences, and 5 Bactrian camel VHH sequences equipped with heavy chain gene promoters, signal sequence exons, and signal sequences for recombination. The single-domain antibodies obtained as a result of immunization of transgenic mice and further selection for affinity to RBD were comparable in affinity and neutralizing ability to both classical mouse antibodies and llama antibodies.
Several groups of scientists[79, 80] have successfully improved the affinity and neutralizing ability of nanobodies by creating multivalent structures. The antibody VHH-72, obtained as a result of llama immunization, was developed in the form of a dimer of variable domains connected by an amino acid linker and in the form of a fusion protein with a constant part of human immunoglobulin IgG1 (VHH-72-Fc). These molecules were dimerized into bivalent complexes, increasing the neutralizing ability of nanobody compared to monomeric variants. The IC50 value of the dimer VHH-72-Fc in the detection system based on pseudotyped vesicular stomatitis virus with luciferase was ~13 nmol L–1[79]. The authors of this study also created fusion proteins of a nanoantibody with a constant part of mouse and hamster antibodies and used computer modeling to increase the affinity of nanoantibodies. Dissociation constant of the initial nanoantibody complex VHH-72 with coronavirus S-protein was 1.2 nmol L–1, dimeric proteins showed values of this parameter in the subnanomolar range. Modified dimeric complexes had neutralized SARS-CoV-1 and SARS-CoV-2 in vitro with a subnanomolar value of IC50 and had a protective effect against several variants of SARS-CoV-2 in transgenic mice and hamsters[80].
In the work mentioned above[78], the dimerization of nanoantibodies due to fusion with the constant part of antibodies was supplemented by combining several VHH into one polypeptide. The authors selected nanoantibodies obtained by immunization of llamas and ‘nanomouse’ and created two types of structures. In the first one, the VHH was fused with the constant part of the human antibody IgG1, which creates a dimer of the nanoantibody, and in the second one the fusion of three consecutive VHH with the constant part of the human antibody IgG1 through a longer and more flexible linker of llama antibodies. The affinity of monomeric nanoantibodies to the coronavirus S-protein was in the nanomolar range, the transition to the hexamer lowered KD to picomolar values and reduced the value IC50 from several times to two orders of magnitude (the minimum values were in the picomolar range).
One of the key advantages of nanobody compared to classical antibodies is the relative the ease of creating multi-specific recombinant proteins[81]. Koenig et al.[82] immunized llamas and alpacas, obtained nanoantibodies with nanomolar dissociation constants, and identified epitopes for the most affine variants. Using X-ray diffraction analysis, the authors analyzed the effect of binding nanoantibodies on the structure of the coronavirus S-protein in single manner and in combinations. Comparing these data with the structure of the trimeric S-protein, the researchers selected the appropriate linker length and the most successful combinations of nanoantibodies to create fusion proteins. The resulting bifunctional dimers and homotrimers reached picomolar values of dissociation constants and reduced the values of neutralizing activity (IC50) by 12 – 18 times compared with similar parameters of monomers[82]. IC50 values for multimers were in the nanomolar concentration range, and the authors believed that the neutralizing effect was associated not only with the shielding of the S-protein interaction region with ACE2, but also with conformational changes in the S-protein, which did not allow it to induce fusion of virus and cell membranes.
It was shown[83] that the homotrimers of the mNb6-tri nanoantibody retain their avidity and show neutralizing activity in the picomolar range after lyophilization, aerosol spraying and heating to 50 °C. The combination of the stability of nanoantibody multimers with the possibility of their production in bacteria makes these proteins promising components for creating aerosols designed to prevent coronavirus infection. This was confirmed by the group of Xiang et al.[84], who created dimeric and trimeric fusion proteins based on obtained monomeric nanoantibodies with surprisingly high affinity. The designed multimers neutralized the virus in vitro in the picomolar concentration range (from 1.3 to 46.9 pmol L–1), and the introduction of an aerosol form of PIN-21 homotrimer shortly after infection of hamsters with SARS-CoV-2 reduced the viral load and weight loss of animals[85].
Another nanoantibody heterodimer was combined into a single polypeptide with a mouse serum albumin-specific nanoantibody to increase the blood circulation time[86]. This heterotrimer was administered intraperitoneally transgenic mice of the K18-hACE2 line on 1, 3, 5 and 6 days after infection with coronavirus, which made it possible to reduce viral loads and prevent weight loss by animals. Remarkable results were published by researchers from Novosibirsk[87]: using repeated immunization of llamas with different variants of the RBD domain (including the Wuhan variant, SARS-CoV-1, beta, delta and omicron strains), they managed to obtain a panel of highly affine VHH, which were fused with Fc fragments of human antibodies. Such bivalent VHH-Fc had KD values in the range from 10–12 to 3.2 × 10–9 mol L–1 for different variants of RBD protein and had neutralizing activities in vitro in the range from 1 to 1539 μg L–1. It is important to note that among the antibodies obtained by repeated immunization with different VHH variants, antibodies with broad neutralizing specificity prevailed, effective, among other things, against the newest evolutionary omicron subvariants, such as BA.5, B.Q.1.1 and XBB.1.16.
In general, it can be concluded that the entire arsenal of existing methods is successfully used in the engineering of nanoantibodies works with classical antibodies. The created multimeric forms, in their affinity and effectiveness, approach the best values of these parameters for antibodies, and the most successful ones may even exceed them.
Another natural source of highly variable proteins, whose binding domain is formed by a single polypeptide chain, are the variable lymphocyte receptors (VLRs) of cyclostomes (lampreys and hagfishes), discovered at the beginning of the XXI century. Unlike classical immunoglobulins, VLRs are based on leucine-rich repeats that form a concave binding surface complemented by a C-terminal hypervariable loop[88, 89]. The secretory proteins VLRB are analogous to the antibodies of other vertebrates in cyclostomes, and the transmembrane proteins of lymphocytes VLRA and VLRC are analogs of T-cell receptors. Due to the unique geometry, interacting with the antigen surface, VLRB can bind to more extended epitopes compared to classical antibodies. Their independence from disulfide bonds, the possibility of cytoplasmic expression, and stability over a wide range of pH values and ionic strength of the solution make them attractive objects for biotechnology[90].
By the beginning of the COVID-19 pandemic, VLR selection methods had already been worked out, so they were successfully used to produce proteins that neutralize SARS-CoV-2. Jurkat cells fixed with formaldehyde and coated with recombinant S-protein were used for the immunization of lamprey larvae. Two weeks after the first injection, repeated immunization was performed, after that RNA was isolated from the blood of larvae and a library of VLRB coding sequences was obtained, next it was transformed into budding yeast cells. Yeast cells expressing affine VLRs were isolated using magnetic beads coated with recombinant S-protein. Next, the coding sequences were cloned and expressed in human embryo kidney cells (HEK293T), and the VLRB proteins secreted by them were then tested for binding to the surface of HEK293F cells expressing S-protein. The most specifically binding VLRs were tested using a solid-phase ELISA and in vitro tests to neutralize the SARS-CoV-2 virus. Thus, several VLR variants have been obtained that specifically bind to the RBD domain of the SARS-CoV-2 S-protein, but not other related coronaviruses. Two of them, named B7 and B39, blocked the entry of wild-type virus into VeroE6 cells with an efficiency comparable to that of monoclonal antibodies. Thus, the IC50 values were 54.9 and 95.9 μg L–1, respectively (the IC50 value for the monoclonal antibody 4A8 used as a control was 355.5 μg L–1)[20]. Nevertheless, given the immunogenicity of the VLRs themselves, the authors suggested using them for diagnosis, but not as a therapeutic agent.
3.3. Artificial scaffolds and affine polypeptides
In addition to natural humoral immunity proteins, artificial scaffolds obtained using display technologies can be a source of binding modules. The drug based on DARPins, artificial proteins containing ankyrin repeats, came closest to the stage of clinical use[91]. Ankyrin repeats are part of eukaryotic cell proteins that bind to various targets, providing organization of the cytoskeleton and regulation of enzyme activity. DARPins are assembled at the gene level from sequences of 4 – 6 motifs containing 6 variable amino acids within one β repeat and two α-helices, and are selected by phage or ribosomal display for their ability to bind to the antigen[91]. Due to their small size, aggregation stability and simple production in bacteria, DARPins are used in the development of biosensors, as well as the creation of fusion proteins and conjugates for the therapy and diagnosis of various diseases[92, 93].
One of the first known drugs of this series is ensovibep, which consists of three different DARPins sequentially connected by linkers that recognize the trimeric S-protein of the coronavirus, and two more DARPins that bind to serum albumin to increase blood circulation time; its total molecular weight is 85 kDa. The drug suppressed the infection SARS-CoV-2 both in vitro and in vivo, while maintaining its effectiveness against the omicron variant[94]. Single intravenous injections of ensovibep proved to be safe and well tolerated[95]. Based on the results in clinical trials, ensovibep had no significant effect on the recovery of hospitalized patients[96], however, in hospitalized patients with COVID-19 symptoms, the amount of virus released decreased, and the risks of hospitalization and seeking emergency medical care decreased by 78% (See ClinicalTrials.gov ID NCT04828161; https://www.clinicaltrials.gov/study/NCT04828161 (last access 10.06.2024).)[97, 98].
The other two trimers are based on DARPins, FSR16m and FSR22, consist of DARPin modules assembled into a three-ray asterisk due to fragments of fibritin of bacteriophage T4 fused with DARPins[99]. Both proteins bind to RBD in the area of interaction with ACE2, neutralize the virus in vitro in nanomolar concentrations, comparable to those for a mixture of monoclonal antibodies REGEN-COV (casirivimab/imdevimab) and other similar drugs[24]. Trimerization of DARPins increases their the neutralizing activity by 300-fold compared to that for monomers. Interestingly, the researchers 24 tested the effectiveness of the drugs obtained by injecting them intranasally to transgenic mice infected with SARS-CoV-2. The use of FSR16m reduced weight loss in the infected mice, as well as reduced viral load in their respiratory tract and the level of proinflammatory cytokines in lung tissues. Thus, this DARPin trimer is a prototype drug that could be used as part of routine or post-exposure prophylaxis against coronavirus without the need for injections.
During the selection of phage display from libraries, other molecules were found that showed their effectiveness in vitro. These include affibody, small proteins weighing about 6.5 kDa, obtained on the basis of StaphylococcuS-protein A.22[100], Affibody neutralizing concentrations analyzed in vitro demonstrates the nanomolar range.
It should be noted that display technologies have made it possible to modify classical antibodies and create from them another type of scaffold based on variable fragments of heavy chains of human immunoglobulin (VH). These proteins, as well as single-domain antibodies of camelids, form an antigen-binding site within a single polypeptide chain and can be selected by phage or ribosomal display. However, this molecules show less immunogenicity, since the framework is formed from the human immunoglobulin heavy chain gene IgG1[101]. VH domains obtained from a synthetic library using a phage display bound to the SARS-CoV-2 S-protein with nanomolar affinity. To increase affinity and time in blood circulation, the VH domains were combined with the Fc-fragment of the IgG1 antibody. Such bivalents neutralized in vitro the virus isolated from the COVID-19 patients[102] and reduced the viral load in hamsters and mice after infection with the original SARS-CoV-2 or its modified variant binding to mouse ACE2[103].
Like other single-domain binding proteins, VH can be combined into multivalent and bispecific constructs, which was implemented[21]. The authors set out to obtain VH binding to the interaction region of RBD and S-protein. To do this, each stage of selection in the phage display began with incubation of a phage mixture with a complex of recombinant Spike-RBD-Fc and ACE2-Fc proteins on beads. Beads were then removed and the remaining phages were selected for binding to Spike-RBD-Fc. Thus, the authors conducted a negative selection of phages for binding to S-protein epitopes not involved in interaction with ACE2, and a positive one for their ability to bind to the RBD contact area interacting with angiotensin convertase. The authors selected three types of VH binding to two different sites within RBD, with dissociation constants of 23 – 113 nmol L–1 and created on their basis multimers. These were homodimers (VH2), homotrimers (VH3), as well as heterodimers connected by linkers, and homodimers assembled from VH fused with the constant part of the antibody (VH-Fc). Multimerization increased the affinity of VH to the recombinant S-protein, KD values reached 0.1 – 8.4 nmol L–1, and the dissociation constant of the VH trimers and the trimeric S-protein ectodomain was 1 × 10–10 nmol L–1. The neutralizing ability of proteins also increased with the transition to multimers: values IC50 varied from ~ 50 nmol L–1 to hundreds of pmol L–1 with heterodimers being more effective than homodimers and trimers are more effective than dimers.
In addition to the direct use of display technologies, preliminary computer modeling was used to develop antiviral proteins. With this help, miniproteins were created based on the spiral–turn–spiral motifs having molecular weight 4 – 7 kDa. The affinity of the miniproteins selected in silico was increased by mutagenesis and yeast display. The dissociation constant of these proteins was in the nanomolar range, and the neutralizing concentrations ranged from 24 to 35 nmol L–1[25]. In the next stage these proteins were modified as follows: the third helix was shortened, and the amino acid residues in the remaining helices were optimized for dimer formation. The resulting dimer, consisting of 4 alpha helices, bound to the S-protein, leading to the formation of S-protein dimers in a head-to-head orientation. Miniprotein dimers neutralized the virus in vitro in the nano- and picomolar concentration range and, when administered intranasally 8 hours before infection, protected Syrian hamsters from weight loss[26].
Another bioinformatic approach was based on the fact that S-protein binding peptides can be selected based on the results of the analysis of the S-protein complex and its natural partner angiotensin convertase, taking the amino acids of the synthetic peptide so as to reproduce the interface of interaction with ACE2. Unfortunately, the size of synthetic oligopepids is limited to the size of 22 – 25 amino acid residues; however, this limitation can be overcome by the formation of disulfide bridges in chimeric peptides. Bibilashvili et al.[104] described two new 5.4 kDa chimeric peptides with affinity for S-protein with KD values in the range of 0.9 – 12 mmol L–1, but an attempt to evaluate it neutralizing activity failed. Verification of a number of previously described oligopeptides aimed at S-protein showed that their affinities (KD) are in the range from 1 to 28 mmol L–1, and the neutralizing activity is not determined. At the same time, covalent crosslinking of peptides after synthesis can improve their neutralizing activity to IC50 = 18 – 25 mmol L–1[105], which is significantly inferior to scaffold-derived peptide analogues. In more detail, the oligopeptides binding S-protein are critically reviewed in the review by Krut’ et al.[106]
The most serious limitation for the therapeutic use of affine proteins that are not human antibodies has become their own immunogenicity. However, scaffolds derived from DARPins and VH proteins are low-immunogenic since they are based on human protein sequences. A significant advantage of such scaffolds is the possibility of increasing avidity due to oligomerization and ease of ‘molecular tuning’, that is the addition of a variety of effector domains to affine modules to form multifunctional therapeutic agents. Such antiviral protein molecules can work in vivo in parallel with the usual immune response, regardless of the patient’s immune status.
3.4. Multifunctional agents based on targeted proteins
Thus, although many different high-affinity proteins that bind to the S-protein and neutralize the virus in vitro and even in animal models have been produced, their clinical trials have not provided sufficient evidence that the neutralizing activity of these proteins is sufficient to completely suppress the infectious process. Apparently, use of such drugs after the onset of symptoms in the patient offers little advantage in the case of rapidly replicating viruses and is mainly capable of preventing viremia and lesions in other organs outside the site of primary viral replication. Most likely, the capabilities of high-affinity molecules that prevent the virus from entering the cell have already been fully used. However, one can imagine agents that enter the cell along with the virion, suppress the infection and enhance the second function of antibodies — their cytotoxicity towards infected cells. The combination of targeted proteins, primarily antibodies, with additional effector modules has been successfully used in oncology, and such a strategy may be useful in the development of similar approaches for antiviral therapy. The target of such a bifunctional agent can be the pathogen genome: the SARS-CoV-2 capsid contains a (+)-RNA genome that replicates without a DNA intermediate.
By the time the COVID-19 pandemic began, several types of RNA-targeting molecules had already been created, primarily specific to the influenza virus genome, including small molecule inhibitors, interfering RNAs, enzymes, and catalytic nucleic acids[107]. Most versatile and least dependent on sequence and secondary structure genome agents were ribonucleases — stable enzymes that cleave RNA, which have already shown their effectiveness against RNA-containing viruses in vitro and in vivo. The ability of bacterial, pancreatic, recombinant and synthetic peptide RNases without targeting modules to suppress the replication of the influenza virus was demonstrated in cell cultures[108-110] and in animals, including intranasal administration of ribonuclease[111]. The binase enzyme was also used against the rabies virus and improved the survival of laboratory animals after infection[112, 113].
Targeted agents specific to SARS-CoV-2 were created based on antibodies and barnase ribonuclease. This monomeric stable enzyme and its inhibitor barstar are currently being successfully used in nanotechnology and experimental oncology[114]. In this case, the authors used the ribonuclease activity of barnase to enhance the antiviral effect of the P4A1 antibody[115]. Conjugation of the antibody with liposomes containing barnase increased the neutralizing activity of the complex by 40 times in the pseudotyped lentivirus model compared to that of an unmodified antibody[116]. Successful conjugation of barnase itself with the P4A1 antibody bearing a motif for enzymatic conjugation by sortase A at the C-end of a heavy chain was also performed[117]. Barnase is a bacterial ribonuclease that can be secreted into the periplasm. In this case, cytoplasmic RNA molecules are protected from barnase, accidentally synthesized on free ribosomes, by the protein inhibitor barstar[118]. This expression system makes it possible to create fusion proteins with barnase and produce them in bacteria[119, 120], which in the future can be used to create bifunctional virus-specific proteins based on barnase and a compact targeted protein.
4. Conclusion
The COVID-19 pandemic began at a time when there were already many platforms for creating targeted peptides and proteins. The accelerated development of drugs simultaneously with the spread of the virus and its mutated variants forced researchers to use all possible technologies and revealed the weaknesses of various approaches. It can be concluded that the available platforms have made it possible to create high-affinity proteins that specifically bind to the S-protein of the coronavirus. The greatest affinity was achieved in the development of classical antibodies, nanobodies, and combinations of several binding modules into multivalent structures with high avidity.
At the same time, a direct comparison of the effectiveness of the obtained agents is complicated by the fact that they were tested on different systems and against different strains of SARS-CoV-2 or pseudotyped virus. As for the most significant indicator, clinical efficacy, data on this parameter are available only for antibodies and DARPin-based constructs. These drugs had a very modest effect in the case of severe patients, but significantly reduced the likelihood of severe disease in ambulatory patients. To date, no drug based on proteins binding to the surface of the SARS-CoV-2 virion has a current approval for clinical use, since none of these agents has proved to be sufficiently effective in vivo.
1) Neutralization with exogenous antibodies or polypeptides may be ineffective due to biological peculiarities of the virus. The SARS-CoV-2 coronavirus multiplies primarily on the mucous membrane of the respiratory system, at least at the start of the infection. Most of the antibodies tested in vivo were of the IgG isotype; constant parts of the same type were used in fusion proteins. IgG antibodies circulate in the blood for a long time but accumulate weakly in the mucous membranes. For instance, after intravenous administration of antibodies of the IgG isotype, their concentration in the lungs is 200 – 500 times lower than the concentration in the blood serum[121]. In addition, the virus enters the cells of the respiratory epithelium through the apical membrane, while virus-binding proteins administered intravenously act from the side of the basolateral membrane, which is separated from the lumen of the respiratory tract by tight junctions before the development of inflammation.
2) The virus is mutating, and new variants have emerged faster than new drugs have been developed. S-protein mutations reduce the neutralizing ability of therapeutic proteins. The solution to this problem may be to use the most conservative protein motifs as targets. At the same time, practice has shown that mixtures of several proteins can be more effective than monopreparations.
3) Virus-specific proteins are mainly considered as therapeutic rather than prophylactic agents. However, the symptoms of the disease and the possibility of detecting the virus appear only after the virus penetrates the cells, where it becomes practically invulnerable to proteins that block its binding to the cell. In addition, the virus is shown to possess cell-to-cell transmission, protecting it from neutralization by antibodies as it spreads throughout the body[44, 122]. Apparently, injected antibodies and fusion proteins with the constant part of antibodies, like antibodies formed in response to infection or vaccination, limit infection mainly due to the attraction of the immune cells due to the constant part. It should be noted that a decrease in the severity of the disease treated with antibodies and other proteins may be associated with a decrease in the likelihood of viremia and multiple organ damage.
Overcoming these difficulties has already led to the development of strategies that increase the effectiveness of virus-specific proteins, and the search for new solutions is likely to continue. Thus, in order to preserve the high affinity of binding proteins to the virus, it is possible to find the most conservative epitopes, as well as create mixtures of several proteins and multimeric structures recognizing several epitopes. For better accumulation of antibodies in the mucosa, various antibody isotypes are selected and their intranasal administration is used[18]. Finally, the binding of the agent to the proteins of the virus is accompanied by exposure of the infected cells to the constant part of antibodies or the introduction of molecules toxic to the virus or infected cell.
In general, the injection into the bloodstream of proteins that bind specifically and with high affinity to the S-protein SARS-CoV-2 can supplement existing humoral immunity, but for the drugs developed to date, this contribution is difficult to call decisive. Most patients with COVID-19 produce their own antibodies; in addition, the cell-based immunity turns out to be at least as important as the humoral one[123].
Interesting results were obtained[124] on a model of ACE2-humanized mice unable to produce antibodies due to deletion of the D-segments of the IGH locus. These mice retained B lymphocytes, but completely lacked antibodies; at the same time, due to human angiotensin convertase, they could be infected with SARS-CoV-2. It turned out that the viral load and clinical picture of the disease in such humoral immunodeficient mice did not differ from similar indicators for immunocompetent ACE2-humanized mice. Moreover, mRNA vaccine vaccination was effective in both groups of mice, leading to the same activation level of specific activated T cells producing TNF and IFNγ. Based on the data obtained, Fumagalli et al[124]. suggested that T cell immunity is completely sufficient to protect against SARS-CoV-2, and humoral immunity does not play a significant role in this process. Indirectly, this observation is confirmed in humans: five patients with multiple sclerosis who received the anti-CD20 antibody ocrelizumab, which depletes B cells, recovered from COVID-19 with minimal titers of specific antibodies[125]; out of ten COVID-19 patients with antibody deficiency caused by common variable immunodeficiency (CVID) only one required hospitalization[126].
In light of the data on the predominantly T-cell control of COVID-19 disease, the combination of the success of high-affinity SARS-CoV-2 antigen proteins in in vitro tests and low efficacy in clinical practice seems quite logical. Unfortunately, no recombinant proteins or complexes equipped with additional effector modules have reached clinical trials, which makes it impossible to compare their efficacy with classical antibodies. Perhaps evolutionary trends toward shortening the infectious cycle and decreasing immunogenicity of SARS-CoV-2 virus would have reduced the efficacy of these proteins and complexes to the same extent. We hypothesize that drugs based on targeting proteins may find application in long-standing or chronic viral infections, such as viral hepatitis.
The experience gained in developing therapeutic agents against coronavirus will undoubtedly be useful for the development of effective targeted drugs to combat known and novel viral infections.
5. Abbreviations
а.a. — amino acid residue,
ACE2 — angiotensin-converting enzyme 2,
CHO — Chinese hamster ovary cells,
COVID-19 — new coronavirus disease, Coronavirus disease 2019,
CVID — common variable immunodeficiency,
ELISA — enzyme-linked immunosorbent assay,
Fc — fragment crystallizable region of antibody,
FDA — The United States Food and Drug Administration,
IC50 — half-maximal inhibitory concentration,
IgG — G-isotype antibody,
IL-n — interleukin n,
NK — Natural killer cells,
Re — effective reproduction number of the virus,
R0 — basic reproduction number of the virus,
SARS-CoV-2 — Severe acute respiratory syndrome-related coronavirus 2,
SPR — surface plasmon resonance,
TNF — tumor necrosis factor,
VH — variable domain of antibody heavy chain,
VHH — variable domain of camelids antibody heavy chain,
VLR — variable cyclostomes lymphocyte receptors,
WHO — World Health Organization.
Acknowledgements
The review was prepared with the financial support of the Ministry of Education and Science of the Russian Federation under Agreement No. 075-15-2021-1049.
References
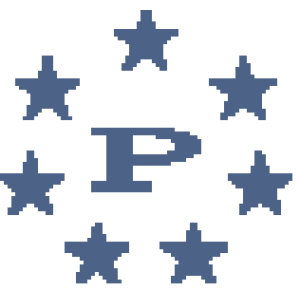
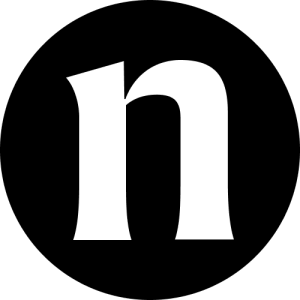
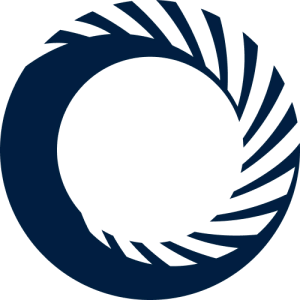
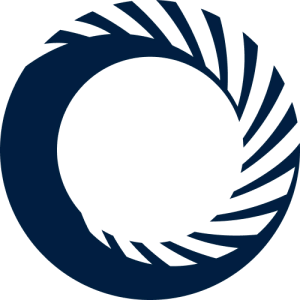
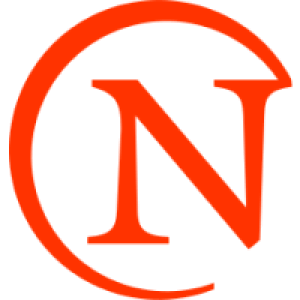
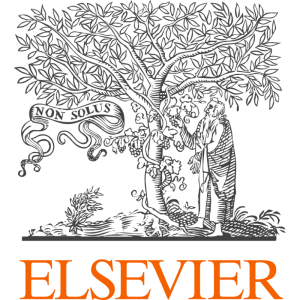
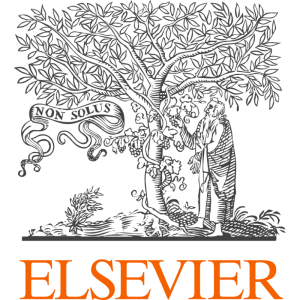
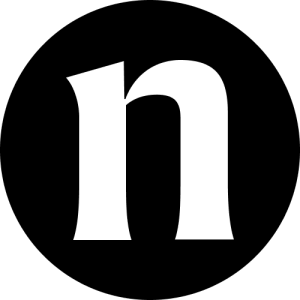
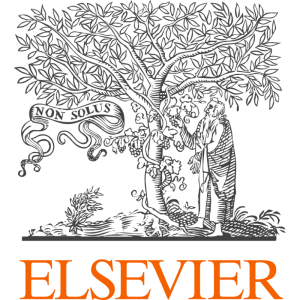
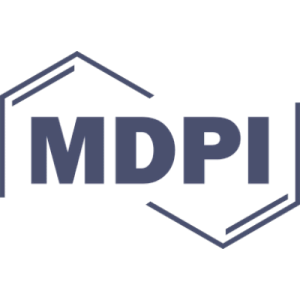
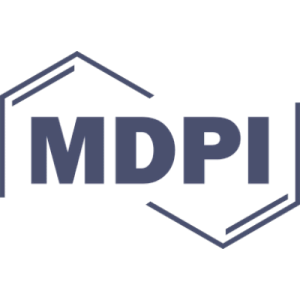
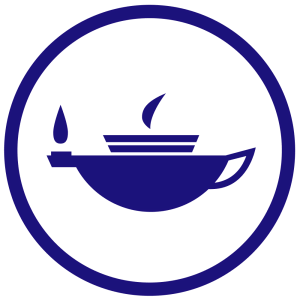
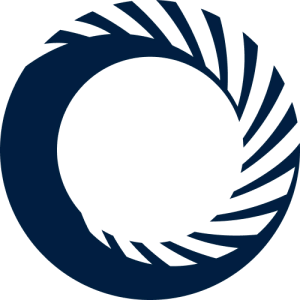
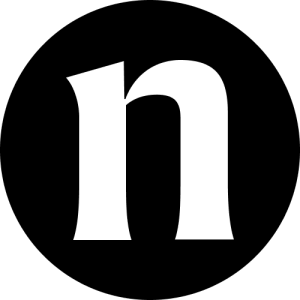
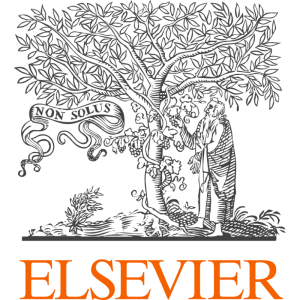
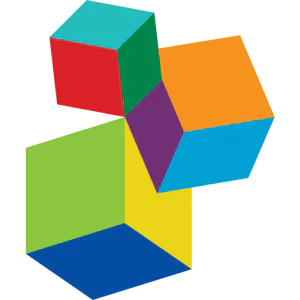
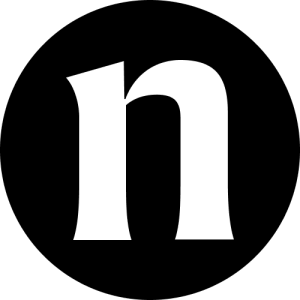
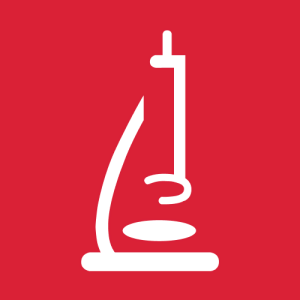
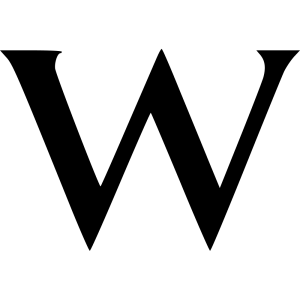
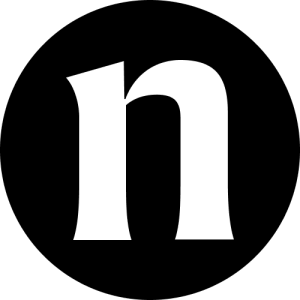
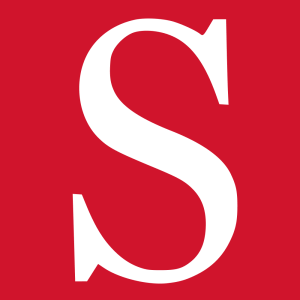
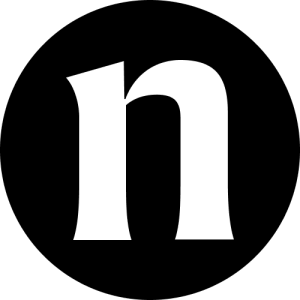
Immunologiya po Yarilinu (Immunology According to Yarilin) (Moscow: Izd. GEOTAR-Media)
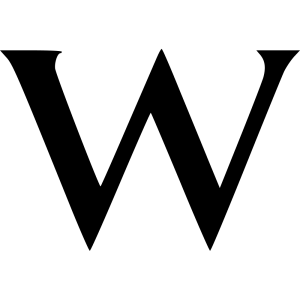
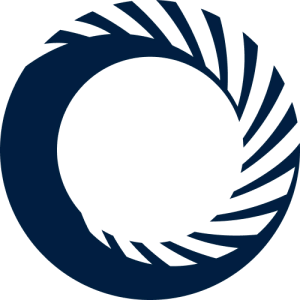
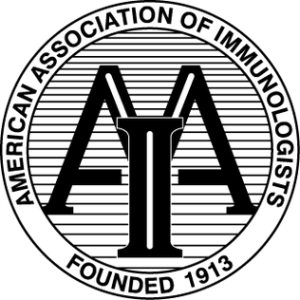
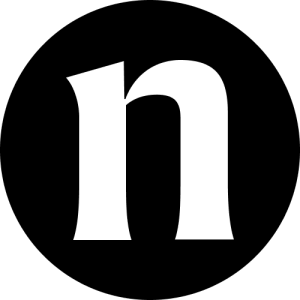
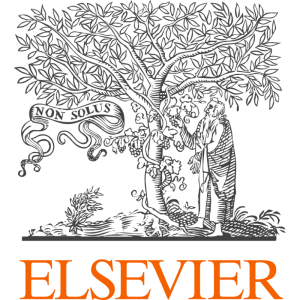
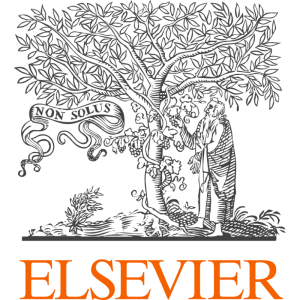
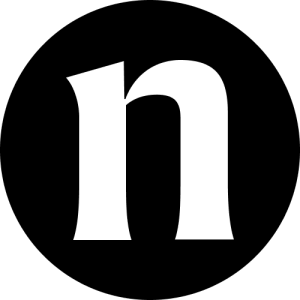
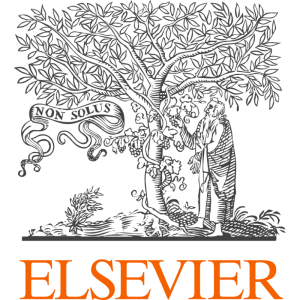
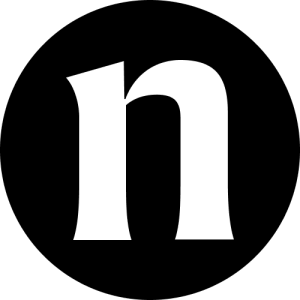
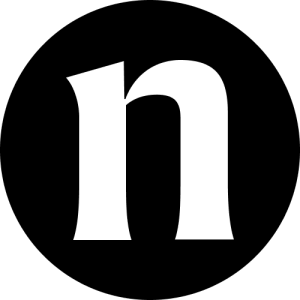
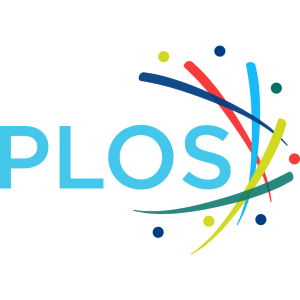
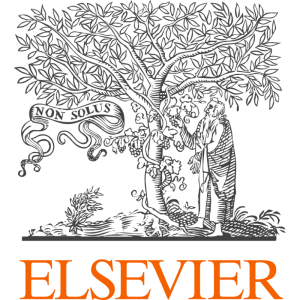
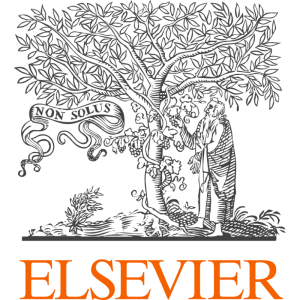
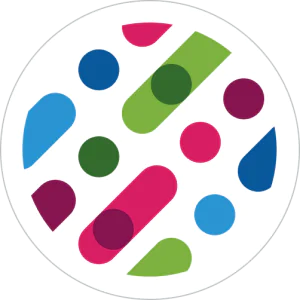
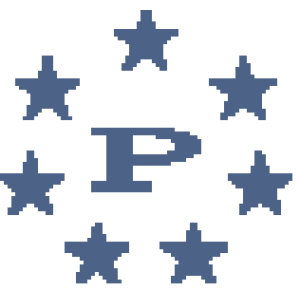
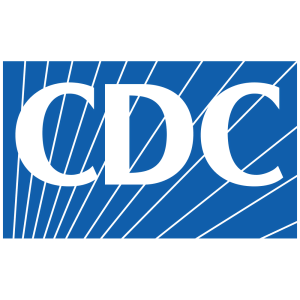
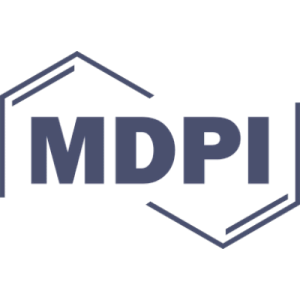
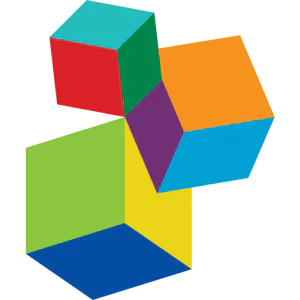
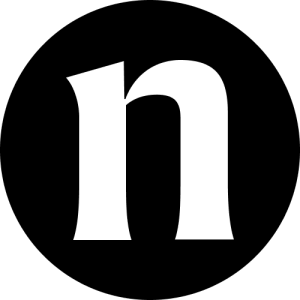
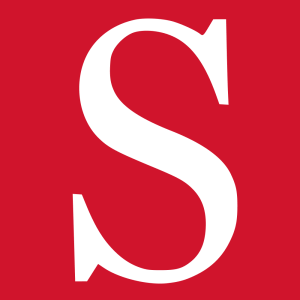
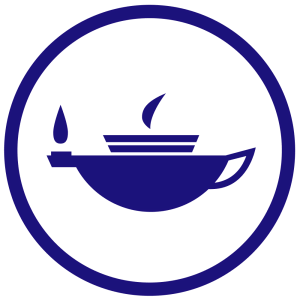
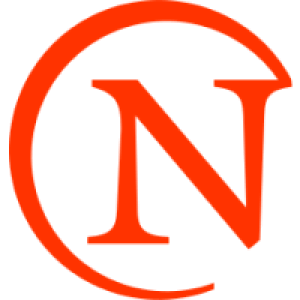
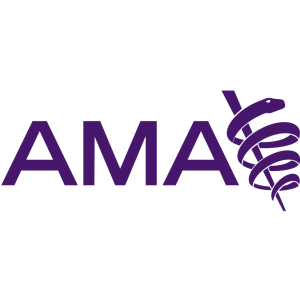
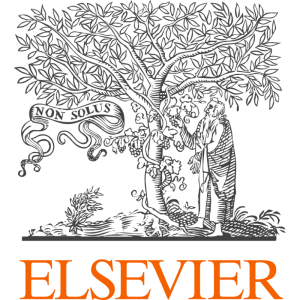
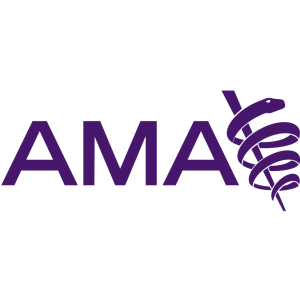
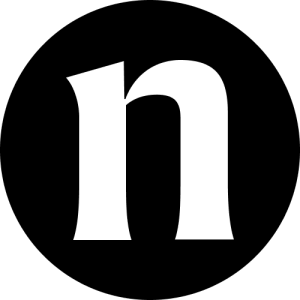
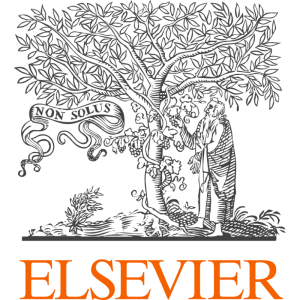
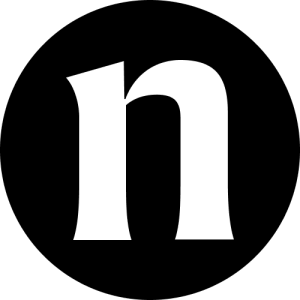
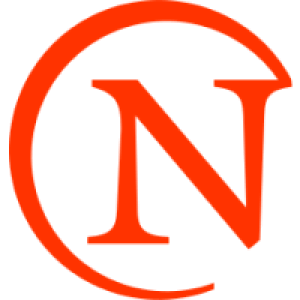
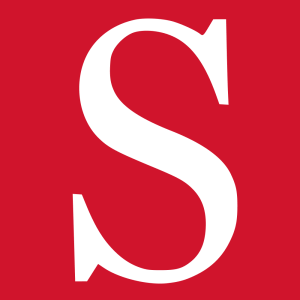
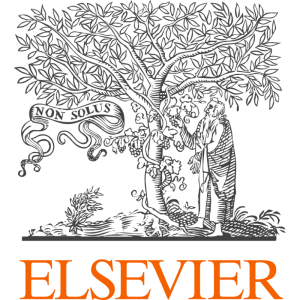
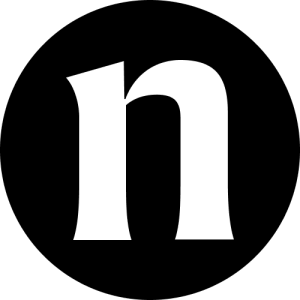
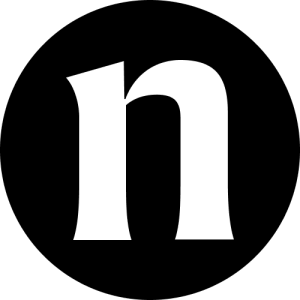
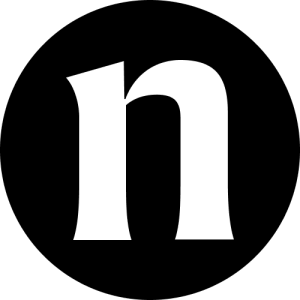
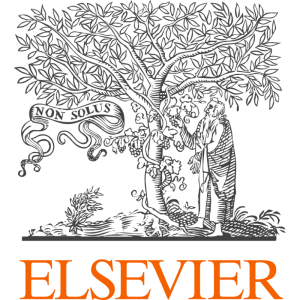
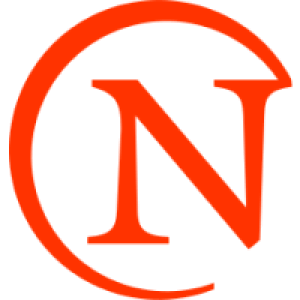
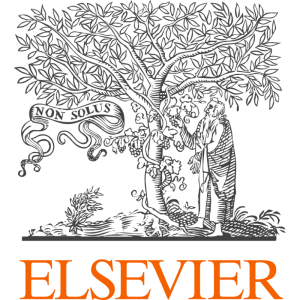
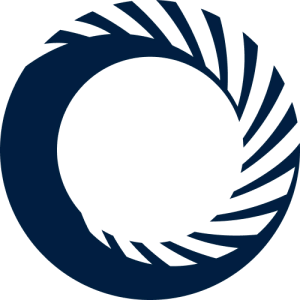
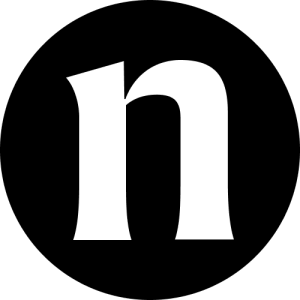
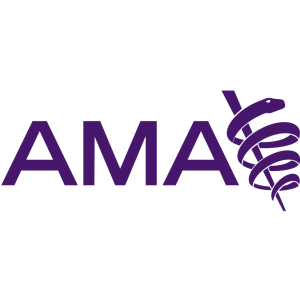
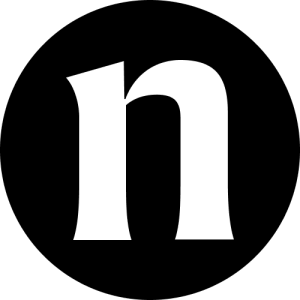
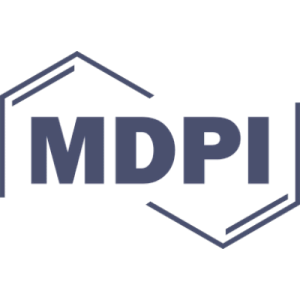
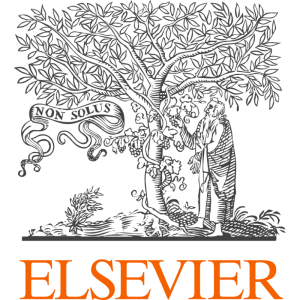
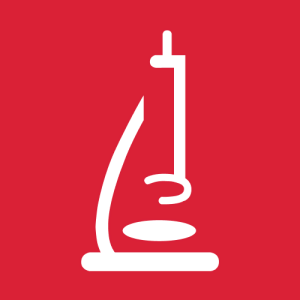
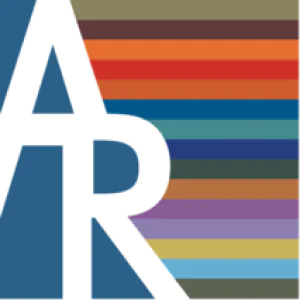
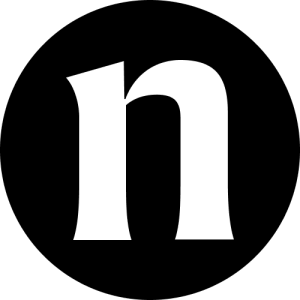
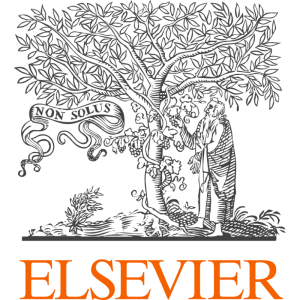
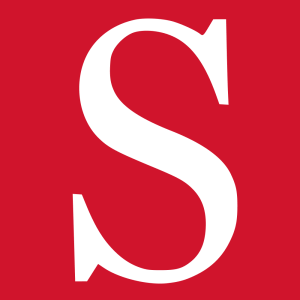
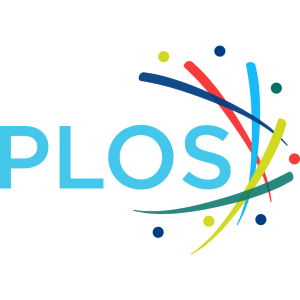
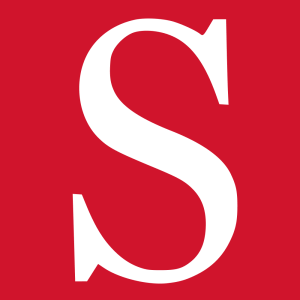
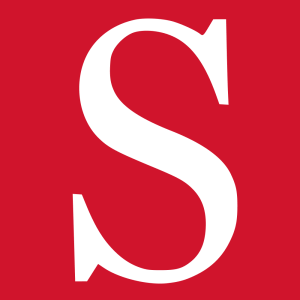
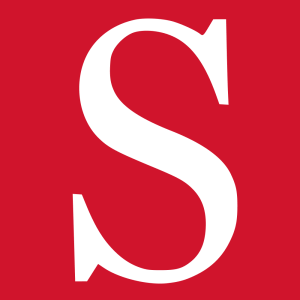
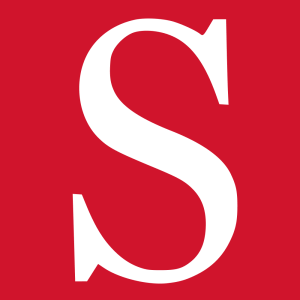
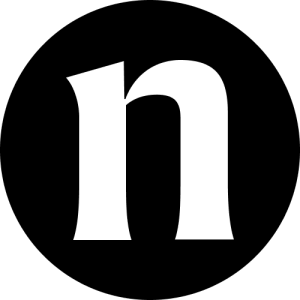
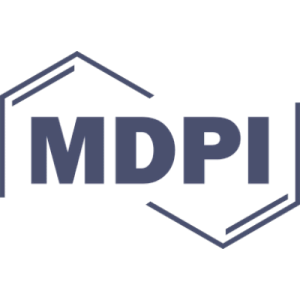
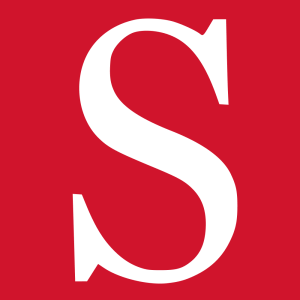
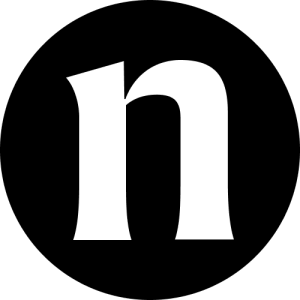
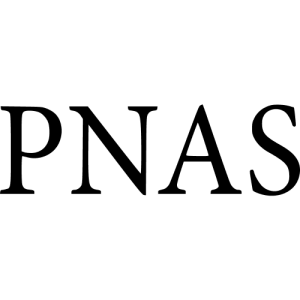
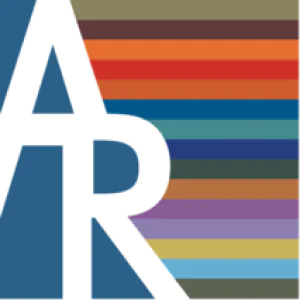
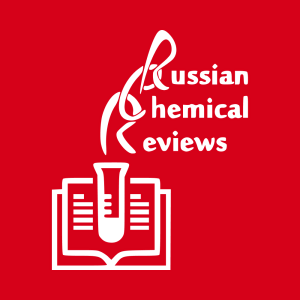
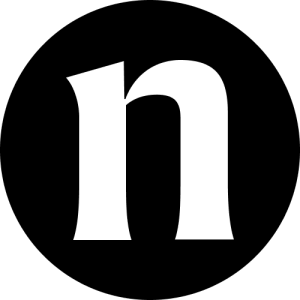
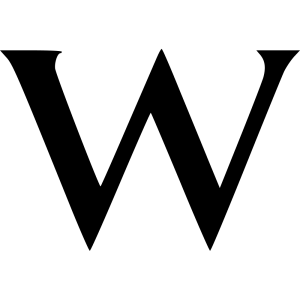
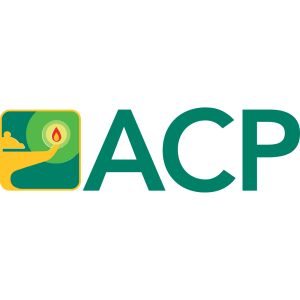
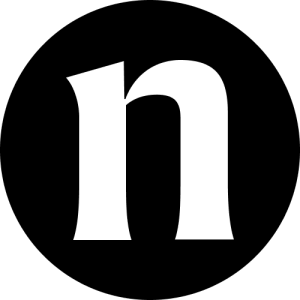
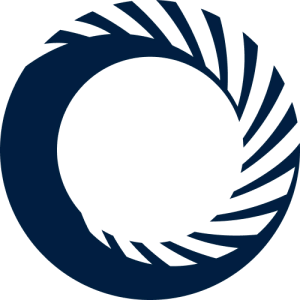
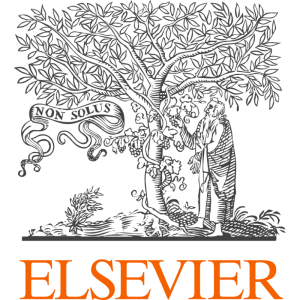
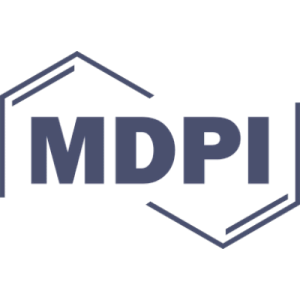
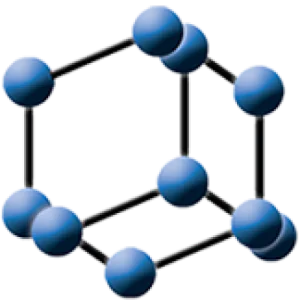
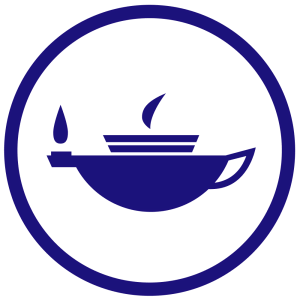
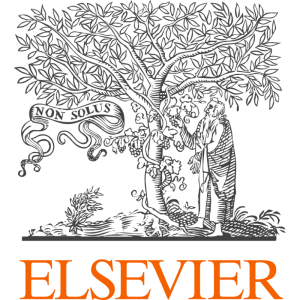
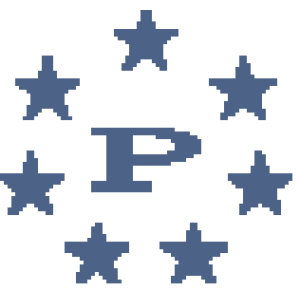
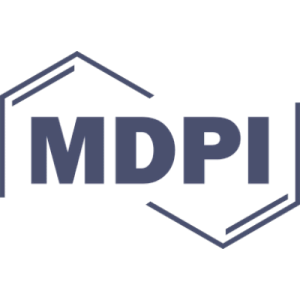
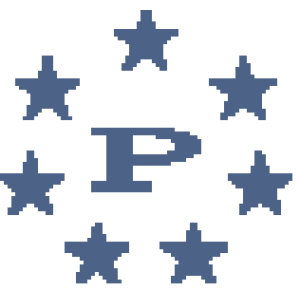
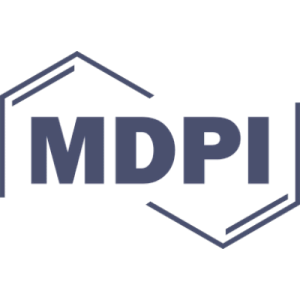
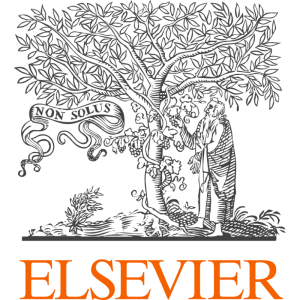
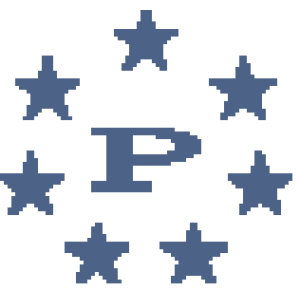
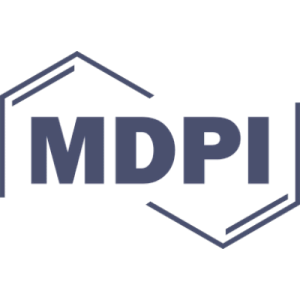
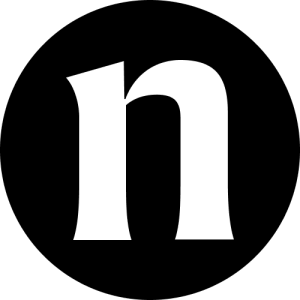
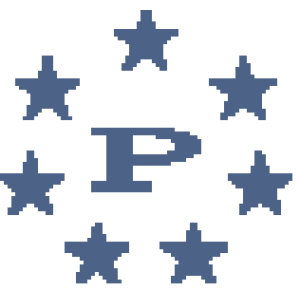
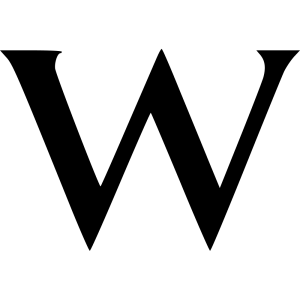
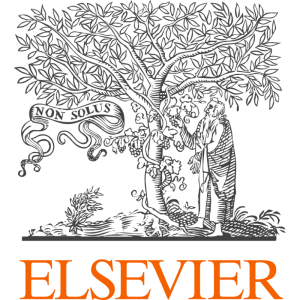
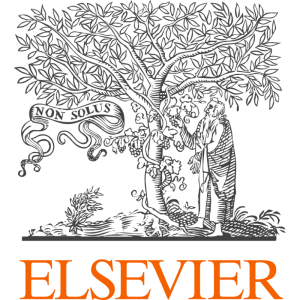
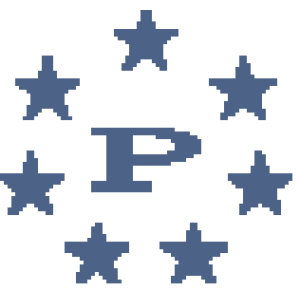
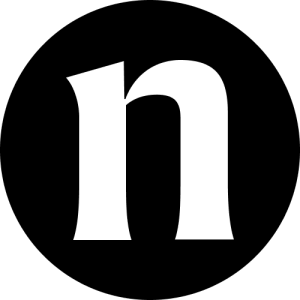
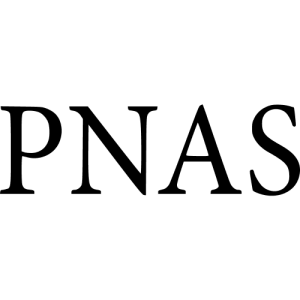
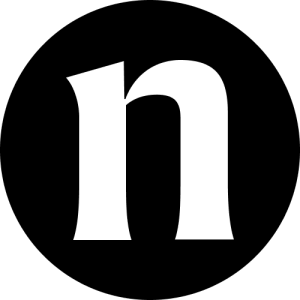
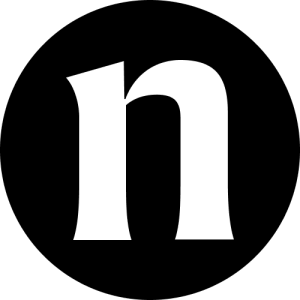
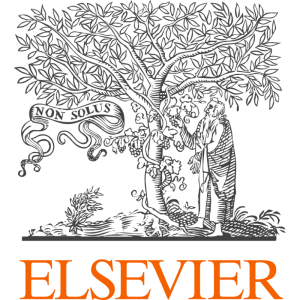
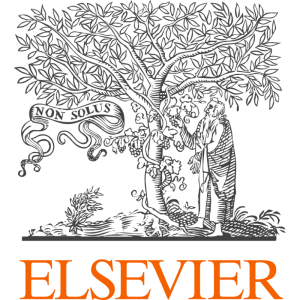