Keywords
Abstract
The review integrates and systematizes data published in the last 15 years on the physicochemical characteristics of specific chemical compounds formed by metal elements with nitrogen atoms containing three or more nitrogen atoms per metal atom. Most often, the total number of nitrogen atoms exceeds their greatest number allowed by the formal higher oxidation state of the metal atom present in the compound. The conceptual possibility of practical application of these compounds now and in the future is also discussed.
The bibliography includes 230 references.
1. Introduction
Nitrogen is known to be one of the most important chemical elements, first of all, because of its predominance in the Earth’s atmosphere (78.3 vol.%) and the presence in living organisms as the key component of proteins and nucleic acids[1]. Like carbon, which is a nitrogen neighbour in the Periodic Table of Elements, nitrogen is able to form relatively long chains consisting of only nitrogen atoms, which can be both closed and open. According to the results of numerous theoretical studies that are described in original publications (see, for example, Refs[2-11]) and reviews[12-14], neutral polynitrogens composed of 4 to 120 atoms can, in principle, exist; examples of their molecular structures are presented in Figure 1. However, it should be noted that currently, the existence of only one such compound, namely, tetranitrogen N4 with a regular tetrahedral geometry, was reliably established experimentally (Figure 1a)[15, 16]. It is beyond doubt that increase in the number of nitrogen atoms in polynitrogen molecules would be accompanied by a decrease in the thermodynamic stability (at least, because any of these compounds will be prone to degradation by the pathway N2n → nN2, and the standard entropy of this reaction increases with increasing n), which is supported by the results of quantum chemical calculations. According to reported data[12-14], there is still little hope that these polynitrogens could be preserved for a relatively long time after they are formed. Nevertheless, polynitrogens by themselves are of considerable interest as potential high energy density materials (HEDMs), which can be practically used to develop new-generation explosives[17].
A promising way to attain stabilization of the polynitrogen molecular structures is to convert them to metal nitrides, which are also high-energy materials, but generally much more stable than their polynitrogen counterparts. In this regard, it is noteworthy that metal nitrides are more useful than non-metal nitrides for the stabilization of polynitrogens, because, unlike the latter, metal nitrides can be formed, in some cases, upon the direct combination reaction of nitrogen with metals at high temperatures and/or high pressures. Furthermore, a chemical and heat treatment process long known in metallurgy is nitriding[18], in which the surfaces of various metals or alloys are saturated with nitrogen in a special nitriding environment. After this treatment, the metal surface acquires enhanced corrosion resistance, fatigue strength, low friction and, what is especially important, high microhardness, which much exceeds the microhardness of the untreated metal. These changes are related, in one way or another, to the formation of various metal nitrides. Meanwhile, in the case of non-metals, as can be easily noticed even from general considerations, nitriding presents no practical interest. Finally, metals evidently represent the majority of chemical elements; all the above facts give reasons to consider particularly metal nitrides in detail.
The range of compounds of nitrogen with metals is fairly extensive; therefore, before considering them, it would be appropriate to develop a systematization of these compounds (which is still lacking), being guided by the rules of the modern IUPAC nomenclature. From a purely formal point of view, all these compounds can be called metal nitrides, or metallonitrides, because nitrogen, being the third most electronegative chemical element after fluorine and oxygen (the Pauling electronegativity of nitrogen is 3.0), has a negative oxidation state in any compound with a metal. Nevertheless, one should distinguish between the terms metal nitrides used in the broad and narrow senses. In the broad sense, this term refers to any chemical compound that consists of atoms of nitrogen and any metal (both present in any number). Meanwhile, in the narrow sense, this refers only to compounds that contain nitride anions N3– (for example, Na3N or Ba3N2). Therefore, we would like to note that in our discussion, we use the term ‘metal nitrides’ particularly in the broad sense. The major part of these metal nitrides are chemical compounds containing both metal–nitrogen and nitrogen–nitrogen bonds. In this case, however, the systematization of these compounds is somewhat complicated because of the diversity of criterial features that can serve as the basis for systematization. In the first approximation, they can be divided into two large classes: (1) metal nitrides the composition of which formally corresponds to the valence ratio of nitrogen and the metal M bound to nitrogen (Co3N2, AlN, Ti3N4) and (2) metal nitrides in which the metal to nitrogen ratio does not correspond to valence requirements (CuN5, YN11, Ta2N8). Other approaches to systematization are also possible; for example, in terms of the number of metals in a structural unit, mononuclear and polynuclear compounds can be distinguished. The latter can be classified into polyhomonuclear and polyheteronuclear. This classification can be further developed by distinguishing so-called homometallic metal nitrides, which contain atoms of only one metal apart from nitrogen, and heterometallic ones, which contain atoms of at least two different metals apart from nitrogen atoms.
The structural units of metal nitrides are quite diverse; they can be classified depending on how nitrogen and metal atoms in them are linked to one another. In this respect, it is possible to distinguish acyclic metal nitrides, in which there are no closed rings of atoms, and cyclic ones, in which at least one ring is present. The last-mentioned type can be further subdivided into two groups, one containing only nitrogen atoms in the rings and the other containing both nitrogen and metal atoms in the rings. Examples of such compounds are shown in Figure 2. Finally, metal nitrides can be assigned, in terms of the ground-state electron configuration of the metal atom, to one of four types (s, p, d or f ). In our opinion, this subdivision of metal nitrides is most adequate for further consideration. Therefore, the material related to these compounds, in one or another way, is presented according to this classification. In addition, the discussion will take into account the above-mentioned classification into homometallic and heterometallic metal nitrides.
The first metal nitrides, namely, barium Ba(N3)2, silver AgN3 and mercury Hg2(N3)2 azides were prepared back in 1890[19]; since then, investigation of compounds of this type has been in progress. Particularly metal azides, containing the –N=N≡N structural group, were among the first and simplest HEDMs. In the first half of the 20th century, quite a few studies addressing these compounds have already been published; the information on these publications can be found in a review[20]. It should be noted, however, that up to the end of the 20th century, studies in the field of physicochemistry of nitrogen compounds with metals were actually limited to azides and mononuclear nitrides (see, in particular, Refs [21-25]). Actually any comprehensive studies of those metal nitrides that, first, contain more than three nitrogen atoms per one metal atom and, second, do not belong to the class of azides (i.e., contain nitrogen moieties other than the azide ion) was started only in the early 21st century. This was promoted by the emergence of a new promising research area at the border of physics and chemistry: high-pressure physicochemistry[26-31]. The above-indicated compounds of metals with nitrogen can be called, albeit somewhat conditionally, higher metal nitrides (HMNs). The number of nitrogen atoms in these compounds is usually greater than their maximum number expected according to the formal highest oxidation state of the particular metal. Metal nitrides of this type, which are of particular interest for solving the problems of both stabilization of polynitrogens and improvement of the nitriding processes, form the subject of the present review. The attention of the author is focused on the original publications that appeared in the current 21st century (the more so, because today they constitute the main body of publications dealing with these compounds). There are still no generalizations concerning the physicochemistry of HMNs in the literature; the attention is attracted by two important issues. First, among the published studies that are discussed in this review, the vast majority of studies are devoted to purely theoretical calculations related to higher metal nitrides. Meanwhile, the number of papers in which experimental data are given, apart from the theoretical calculations, is much smaller [it should still be noted that the ratio of the total numbers of theoretical and experimental studies markedly depends on the particular class (s, p, d or f ) of the metal present in the nitride]. Second, along with calculations of molecular structure and/or crystal lattice parameters of HMNs, theoretical studies often include calculations of other physicochemical characteristics (thermodynamic, spectral, mechanical, etc.) for these compounds. A discussion of all these characteristics together when considering any of the publications cited below appears quite difficult if only because of their diversity. Hence, it is more appropriate to discuss them separately. In view of this important circumstance, we will first focus on the structural features of higher metal nitrides (this is the subject of chapters 2 and 3) and only after that, other physicochemical characteristics of these compounds will be addressed, first of all, those characteristics that are directly related to applicability of the nitrides as HEDM components (chapter 4).
2. Homometallic higher metal nitrides
This chapter presents and analyzes the information currently available from the literature concerning specific features of molecular structures and/or crystal lattices of higher metal nitrides with the general formula MnNk formed by atoms of a single s-, p-, d- or f-metal M (n, k are integer numbers). This chapter includes four parts (2.1 to 2.4), which address s-, p-, d- and f-metal-based nitrides, respectively.
2.1. Homometallic higher metal nitrides of s-elements
These compounds are addressed in Refs [32-72]; to all appearances, the greatest attention of researchers is drawn to nitrides formed by lighter s-elements, that is, lithium[32-40] and beryllium[53-58]. Among other reasons, this may be attributable to the fact that alkali metals, except for lithium, do not react with elemental nitrogen either at ambient conditions or at elevated temperature, which necessitates the search for alternative ways to synthesize their nitrogen compounds. There is also a piece of published data dealing with HMNs of other ns1- and ns2-elements: sodium[41-43], potassium[44-46], rubidium[47-49], cesium[50-52], magnesium[59-63], calcium[63-69], strontium[63, 68, 69] and barium[68-72]. It should be noted right away that most of the mentioned studies devoted to HMNs are mainly theoretical; some experimental data can be found only in a small number of publications[43, 34-40, 45-47, 50-52]. Characteristically, all these studies address compounds of Group 1 s-elements; HMNs of Group 2 s-elements have been investigated only theoretically.
First, we will consider higher lithium nitrides, because, on the one hand, most experimental data available for s-element nitrides refer to particularly lithium nitrides and, on the other hand, owing to the small atomic weight of lithium, these compounds seem a priori to be most promising for the development of HEDMs with high nitrogen contents. In this respect, note first of all a paper by Ge et al.[36], who reported the generation of compounds LiNn and single-charged cations LiNn+ (n = 4, 6, 8, 10) by laser deposition and mass-spectrometric study of the products. The most stable isomers of these compounds (three for LiN4 and four for LiN6, LiN8 and LiN10) were revealed theoretically using the density functional theory (DFT) method; it was stated that transition from LiNn to LiNn+ leads to a fairly pronounced change in the molecular structure accompanied by increase in symmetry (Figure 3). This circumstance was confirmed in a later study[37] also for LiN12 (Figure 4); currently, this compound is the record-holder among metal nitrides in the content of nitrogen (96.03 mass %). It is noteworthy that the structure Li(N2)n/2 is implemented for LiN4, LiN6 and LiN8, while in the case of LiN10 and LiN12, the structures are Li(N2)3(η1-N4) and Li(N2)4(η1-N4), and for their cations only the former structure exists (see Figure 3). The order of the calculated bond energies between lithium and nitrogen atoms corresponds to the order of Li – N2 bond lengths. The N2 structural unit is typical of mononuclear lithium HMNs with an even number of nitrogen atoms; meanwhile, the known higher lithium nitrides with an odd number of nitrogen atoms such as LiN3 and LiN5 comprise different structural groups, namely, azide (N3) and pentazolate (N5) groups, respectively[32-34, 38-40]. Zhang et al.[32] found an interesting high-pressure (P > 35 GPa) phase transition from the azido group N3 to the pseudo-benzene group N6 caused by polymerization of linear molecular chains of azide anions in low-pressure LiN3 molecular phases and by decrease in the phase volume, which leads to closer packing of crystal structural units upon compression. The resulting metastable hexagonal \( P6/m \) phase has relatively pronounced metallic properties[32]. Zhou et al.[38] reported a facile approach to the synthesis of solid lithium pentazolate (LiN5) with the Fedorov space group of symmetry (FSGS) \( P2_{1}/m \) by the reaction of lithium azide LiN3 with dinitrogen at high pressure with laser heating; this compound remained stable at P > 18.5 GPa after depressurization. The bond lengths in the N5 – group are intermediate between the single and double bond lengths[38]. All other ns1-elements form chemical compounds of analogous composition, also containing the pentazolate anion[42, 44, 49, 51, 52]; for sodium, rubidium and caesium, it is actually the only one known metal nitride with the ratio N : M > 3. For potassium, polynuclear metal nitrides, K2N16 (Ref. [44]) and K9N56 (Ref. [46]) were also reported. The structure of the former nitride is composed of a planar two-dimensional extended network of nitrogen atoms forming 18-membered macrocycles; this substance, however, is stable only at P > 70 GPa. The latter compound was obtained by laser radiation-induced direct reaction between KN3 and N2 at high pressure (P > 60 GPa) and at temperatures above 2000 K. The unit cell of K9N56 includes 520 atoms and contains the N64– anion shaped like a planar hexagon[46].
Unlike HMNs of ns1-elements, which typically contain an odd number of nitrogen atoms in a structural unit, ns2-elements more commonly form metal nitrides with even numbers of nitrogen atoms. The simplest example is BeN4 reported in a number of publications[53, 55, 58]. In the earliest publication, Wei et al.[53] predicted the possibility of synthesis of this compound by compressing a mixture of Be3N2 and N2 to a pressure of ~ 40 GPa; the obtained BeN4 crystallizing in \( P2_{1}/c \) FSGS can be stabilized to exist under ambient conditions. This compound contains an extended three-dimensional corrugated polymeric network of N10 rings (Figure 5a). Lin et al.[57] predicted the existence of a beryllium tetranitride BeN4 as a \( P4/nmm \) phase (Figure 5b). Zhang et al.[55] found two other BeN4 phases with extended chains of nitrogen atoms, which were designated in the study as γ- and δ-BeN4. According to thermodynamic data, the γ-BeN4 phase is most stable at atmospheric pressure, while the δ-BeN4 phase is metastable over the whole pressure range (0 – 120 GPa). These phases can be synthesized at pressures of 20.8 and 27.4 GPa, respectively (which is somewhat lower than P = 28.5 GPa, necessary for the synthesis of \( P2_{1}/c \)-BeN4). The reaction of beryllium with nitrogen at P ~ 85 GPa gives one more beryllium tetranitride phase consisting of polyacetylene-like nitrogen chains with conjugated π-systems and Be atoms in a square planar coordination[56]. It is quite possible that HMNs of this s-element with an even higher number of nitrogen atoms may also exist, but there is no relevant information as yet. Other ns2-elements are also quite prone to form tetranitrides MN4 such as MgN4[59-61], CaN4[64, 66] and BaN4[70, 71]. Wei et al.[59] predicted the possibility of existence of MgN4 with \( P\bar{1} \) and \( Cmmm \) FSGSs and MgN3 at high pressures (~ 100 GPa); the authors found that the nitrogen atoms in MgN4 form zigzag-like polymer chains, while in MgN3, they exist as benzene-like N6 rings. For CaN4 and BaN4, two structures with \( P6/mmm \) and \( P4_{1}2_{1}2 \) FSGSs were described; the former structure is similar to the structure of MgN4, while the other one contains N2 groups[64, 70]. The only exception is Sr, for which no data on a tetranitride are available as yet. More nitrogen-rich ns2-element nitrides have also been reported. Examples are Mg2N12 (actually existing as Mg2(N5)2N2[Mg2(N5)2N2]n with N2 groups and N5 – pentazolate anions as structural units)[62], MN6 (M = Ca, Sr, Ba)[68, 69, 72] and MN10 (M = Mg, Ca, Sr, Ba)[63, 67, 70]. In the case of CaN6 and SrN6, the most stable structures crystallize in P1 space group, with nitrogen atoms being connected to zigzag-like polymer chains similar to those in \( P6/mmm \)-CaN4, while in the case of BaN6, the space group is C2/m, with metal ions being linked to the capping pentazolate anions N5(N)–[68]. For compounds MN10, as expected, the most stable structures are metal ion di(pentazolates)[63]; their molecular structures are shown in Figure 6. It is of interest that in the case of M = Sr, both pentazolate groups are located in one plane, whereas for the other three metals M, they are located in mutually perpendicular planes. This fact, however, was not discussed in the cited study[63] or in any other study.
Similar data for CaN10 and BaN10 were also reported in other publications[67, 70]. Huang and Frapper[70] described the binuclear nitride Ba2N11 in which nitrogen-containing structural units are three azide and one dinitrogen groups. The data on the stoichiometric compositions of these compounds are summarized in Table 1. It is noteworthy that most of the publications cited above focused attention on the applicability of these compounds as highly efficient HEDMs (although in some cases, this is at least debatable).
2.2. Homometallic higher metal nitrides of p-elements
The total number of p-elements much exceeds the number of s-elements; however, only a few of them can be classified as metals [particularly four Group 13 (IIIA) elements, three Group 14 (IVA) elements and two Group 15 (VA) elements]. Higher nitrides of these elements have been addressed in a relatively small number of studies[73-92]. Most of these studies are related to nitrides of two Group 13 p-elements, namely, aluminium[73-83] and gallium[81, 83, 85-87]; the nitrides of other elements of this type were mentioned only occasionally[88-92].
In the first publication on this topic, Lee et al.[73] performed a quantum chemical calculation for the molecular structures of polynitrides AlN4, AlN5, AlN6 and AlN7 using DFT method with the B3LYP functional and MP2 and QCISD methods. It was shown that a planar five-membered nitrogen atom ring is the most stable structure for AlN4; a similar planar six-membered structure is most stable for AlN5; and for the two last-mentioned nitrides, non-planar structures of type b shown in Figure 7 were found to be the most favourable. According to another theoretical study[75], which also considered AlN4 and AlN5, the most energetically favourable structure of AlN4 is that where the Al atom is bound to two dinitrogen groups. Subsequently, these compounds were studied only theoretically[74-81]. Yuan et al.[81] noted the possibility of formation of \( P2_1 \)-AlN6 phases and a gallium compound with a similar composition and symmetry at a pressure of approximately 20 GPa; according to this study, M(N2)2 groups are present in the structures of both compounds. The existence of binuclear metal nitride Al2N7 stable at pressures above 30 GPa was also predicted[79]. Running a little ahead in the course of the presentation, we note that gallium also forms higher nitrides of types MN4 – MN7[78, 81-85].
More nitrogen-rich HMNs compared to AlN7, particularly AlN9 and AlN15, have been considered in a number of studies[78, 82, 83]. The first-mentioned compound is aluminium(III) triazide. According to DFT quantum chemical calculations, it has \( C_{3h} \) symmetry[83]; the second compound is tris(pentazolato) aluminium[78, 82]. A similar compound has been predicted for gallium, but, unlike AlN15 for which the \( C222_1 \) FSGS is expected (Figure 7a), in the case of GaN15, the expected space group is \( Cc \) (Figure 7b)[78]. Two more higher metal nitrides with even numbers of nitrogen atoms were predicted for gallium, namely, GaN8 (Figure 8)[87] and GaN10[86], which have no analogues among aluminium nitrides.
The higher metal nitrides of other p-metals have attracted little research attention so far. The most significant study devoted to these metal nitrides is probably that reported by Wang et al.,[89] who considered a number of tin nitrides ranging from SnN3 to SnN20. According to the results of this study, the \( Imm2 \) phase is most stable for SnN3; that for SnN4 has the \( P1 \) space group and contains finite N8 chains; SnN5 has the \( P\bar{1} \) FSGS with finite N8 chains and dinitrogen groups; SnN6 has the \( Ibam \) FSGS; SnN8 has the \( P\bar{1} \) FSGS with oligomeric –N=N–N=N– chains; and finally SnN20 crystallizes in the \( P\bar{4} \) space group with four pentazolate anions per metal atom. There are antimony HMNs: SbN4 (\( C2/m \) FSGS) and SbN9 and SbN15 with trigonal and trigonal-bipyramidal geometry of the nitrogen atoms linked to the metal atom, which are actually nothing other than antimony(III) triazide and antimony(V) pentaazide, respectively[90-92]. The structure of BiN9 considered by Villinger and Schulz[92] is similar to the structure of SbN9, i.e., it is also a metal azide.
Data on the stoichiometric composition of p-element HMNs are summarized in Table 2. The attention is attracted by the fact that, unlike s-element nitrides, the higher nitrides of p-elements were discovered only by quantum chemical calculations at different levels, but have not yet been detected experimentally (although this seems quite probable). The few exceptions are only polyazides SbN9, BiN9 and SbN15[91, 92]. Moreover, for some p-metals, namely, In, Tl and Pb, the higher nitrides have been neither detected experimentally nor considered theoretically.
2.3. Homometallic higher metal nitrides of d-block elements
Most of chemical elements and particularly most metals belong to the d-block; these elements are located in Groups 3 – 12 of the Periodic Table of Elements (or Groups IB to VIIIB in the short form of the Table). These elements are able to form more chemical bonds than s- or p-elements, and, therefore, it can be expected a priori that HMNs with more diverse metal–nitrogen bonds can be obtained for them than for s- or p-elements. In view of the above, it appears quite natural that the number of publications devoted to d-element HMNs much exceeds the number of studies dealing with higher nitrides of s- or p-elements. Currently, higher nitrides have been detected, in one or another way, for all d-elements, except for Tc and Au[28, 78, 93-167]. As in the case of s-element nitrides, a considerable part of these publications give only results of quantum chemical calculations, while the quantity of experimental data on the physicochemistry of these compounds is still minor (although there are more data than can be found for p-elements).
The most pronounced diversity of higher metal nitrides both in the stoichiometry and in the number of publications is inherent in d-elements with the ground state electronic configuration ns2(n – 1)d1 [Group 3 (IIIB)], that is, Sc, Y and La[78, 93-104]. For scandium and lanthanum, only mononuclear HMNs were reported in the literature, whereas in the case of yttrium, both mono- and polynuclear nitrides are known. For Sc and Y higher nitrides, almost all k values from 3 to 10 are encountered, while for La nitrides, only k = 8 and 9 are known. Using the CALYPSO method, the structures of ScN6 and ScN7 existing in the range P = 0 – 100 GPa were predicted[95]. According to the authors, the most stable phases of these compounds have \( P\bar{1} \) FSGS; the former contains a three-dimensional folded multi-nitrogen network (Figure 9a), while the latter contains five-membered N5 rings and N4 units (Figure 9b). ScN6 is thermodynamically stable only at P > 80 GPa, while ScN7 is stable in the range from 30 to 90 GPa. Scandium forms more nitrogen-rich HMNs, in particular, ScN8, ScN9 and ScN11, which were considered theoretically in a recent study[96]. These compounds also have \( P\bar{1} \) FSGS, like ScN6 and ScN7, but they are characterized by more a complex nitrogen atom network (see Figure 9). The greatest k : m ratio is inherent in ScN15[78]. Like AlN15 and GaN15 mentioned above, this is pentazolate M(N5)3, with its FSGS (Сс) being the same as that for GaN15. Like Sc, yttrium also forms HMNs with k from 3 to 10 (except for k = 9); however, their space groups and geometric structures differ from those of analogous scandium compounds. The compound YN6 (\( C2/m \) FSGS) especially stands out due to a unique feature: it has two types of yttrium atoms, one with a coordination number (C.N.) of 18 and the other with C.N. = 13 (Figure 10)[100]; both C.N.s are quite rare for the chemistry of d-elements. Before that study, the cyclic N18 group was not reported in the literature. It is important that this metal nitride and also binuclear Y2N11 have been obtained experimentally by the direct reaction between yttrium and nitrogen at 100 GPa and 3000 K in a laser-heated diamond anvil cell[100]. A unique arrangement of nitrogen atoms, namely, a polynitrogen double helix is also inherent in Y2N11. Using DFT calculations, the dynamic stability of both metal nitrides was confirmed and also a noticeable degree of metallicity, caused by their structural features, was found. Mention should also be made of pentayttrium tetradecanitride Y5N14, which was synthesized, like Y2N11, by direct reaction between yttrium and nitrogen, but under somewhat milder conditions (P ≈ 50 GPa and T ≈ 2000 K)[98]. According to high-pressure single crystal X-ray diffraction data, this compound has \( P4/mbm \) FSGS and contains three types of nitrogen dimers and three types of metal atoms, with C.N.s of 9, 12 and 11.
This makes Y5N14 unique among all known metal nitrides in which nothing of the kind is observed. Y2N11 and Y5N14 possess anion-driven metallicity[98]. According to theoretical calculations, the most nitrogen-rich known yttrium nitride, YN15, has a spatial structure similar to the structure of ScN15[78]. There is only one type of these compounds that is formed by each of three Group 3 (IIIB) metals, namely, MN8 (M = Sc, Y, La): ScN8 has P1 – space group[96], YN8 crystallizes in \( P3_{1}c \) space group[97, 99, 102], and the LaN8 space group is \( Cm \)[104]; in the last-mentioned compound, nitrogen atoms form infinite N∞ chains. Lin et al.[104] reported also compound LaN9 with \( Pm3 \) FSGS in which the metal atoms are bound to N3 groups; therefore, it is actually lanthanum(III) triazide.
The d-element higher nitrides with the ground state configuration ns2(n – 1)d2 [Group 4 (IVB)], Ti, Zr and Hf, were considered in Refs [105-114]. As for Group 3 (IIIB) elements, the greater part of compounds described in these studies are mononuclear MmNk HNMs, where m = 1, and k is an even number varying from 6 to 20. There are four types of higher metal nitrides that are formed by each element of this group, namely, MN6, MN8, MN10 and MN20. For M = Ti and Zr, mononuclear MN12 nitrides are known. For hafnium, this type of nitrides has not yet been reported; therefore, the mere possibility for them to exist is an open question.
The simplest higher metal nitrides of these d-elements, TiN6, ZrN6 and HfN6, were considered only theoretically more than 20 years ago[105]. The molecular structure of each of them has \( C_{6v} \) symmetry and contains planar N64– units coordinated to the metal atom through all six nitrogens similarly to what occurs in arene π-complexes M(C6H6)2 (i.e., η6-N6). However, no new data about these compounds appeared later, and their crystal structure remains an open question; the same is true for TiN8, ZrN8 and HfN8, which were noted to belong to a tetragonal system and to contain infinite armchair-like nitrogen atom chains[110].
The higher nitrides of Group 4 (IVB) elements with a somewhat more complex composition, namely, TiN10, ZrN10 and HfN10 were subjects of a theoretical study[112]. According to the results of this study, these compounds can exist even at relatively low pressure (P < 25 GPa); each has Immm FSGS and contains infinite zigzag chains of nitrogen atoms similar to the chains shown in Figure 8. These compounds also have a very interesting feature, which will be discussed in Section 4.1.
Among the three structures of higher titanium nitride TiN12, with the Ti atom being bound to six dinitrogen groups N2, four azido groups N3, or two hexaazine groups N6, the first-mentioned structure is most stable[106]. This molecular structure belongs to \( D_{3d} \) symmetry, while the second (\( T_d \) symmetry) and third (\( D_{2d} \) symmetry) structures are energetically less favourable by 6.18 and 14.89 eV, respectively. The same energy relationship holds for single-charged ions TiN12+. It is curious that for the first of the above structures, transition from TiN12 to TiN12+ is accompanied by symmetry increase from \( D_{3d} \) to \( O_h \), whereas for the second and third structures, the symmetry decreases (from \( T_d \) to \( C_{2v} \) and from \( D_{3d} \) to \( С_1 \), respectively)[106]. Similar compounds are formed by zirconium with the only difference that the molecular structure of ZrN12 has \( D_{3h} \) symmetry rather than \( D_{3d} \) as TiN12[111]. An absolutely different TiN12 structure containing a 12-membered aza-macrocycle was considered by Mikhailov and Chachkov[109]. It was shown to have \( C_{4v} \) symmetry, with the group of four nitrogen atoms in its TiN4 structural moiety being planar (Figure 11); however, the Ti atom is elevated above the plane of these four nitrogen atoms by 104.4 pm and, hence, the deviation of this moiety from coplanarity is quite significant (67.6°).
Each type MN20 compound (M = Ti, Zr, Hf) has a molecular structure shown in Figure 12a[107]. As can be seen, these compounds, are tetra(pentazolates) of d-metals, as was to be expected. This conclusion was confirmed by Yuan et al.[108], who, in addition, established the existence of TiN20 and ZrN20 as two crystallographically different phases with \( P4/mcc \) and \( I\bar{4} \) FSGSs (Figure 12b,c). Unlike TiN20 and ZrN20, a similar Hf compound was found to have only one stable structure with \( P4/mcc \) FSGS[113]. Hafnium, however, forms a unique tetranuclear nitride Hf4N22, in which the structural groups that constitute the coordination environment of the metal atoms are represented by single nitrogen atoms, dinitrogen molecules and planar chains of four nitrogen atoms, each resembling a boat[114].
The higher metal nitrides for the next d-element group, particular, metals with the electron configuration ns2(n – 1)d3 [Group 5 (VB); V, Nb and Ta] are considered in Refs [109] and [115-122]. These elements are expected to form mononuclear HMNs with both odd and even numbers of nitrogen atoms in the structural units. The available publications (albeit not numerous so far) generally confirm this expectation. However, there is no single type of metal nitrides that would exist for all three above elements. Moreover, there are only two types of nitrides existing for two of the elements: MN4 for V and Ta[118, 122] and MN15 for Nb and Ta[119]. The latter two HMNs are pentaazines with a geometry of regular trigonal bipyramid, the vertices of which are occupied by metal-bound nitrogen atoms. Attempts to prepare a similar compound for vanadium have yet been unsuccessful, judging from the data of Haiges et al.[116], who obtained only salt-like products containing hexa(azido)-vanadate(V) [V(N3)6]–. Nevertheless, the authors experimentally prepared the nitride VN12, which is built as vanadium(IV) tetraazide V(N3)4. A compound of the same composition, but with a completely different structure has been reported[109]; it contains a twelve-nitrogen macrocycle coordinated to vanadium by four nitrogen atoms. Vanadium also forms HMNs with an even number of nitrogen atoms VN4, VN8 and VN10[115, 117, 118], characterized by \( P\bar{1} \), \( P4mnc \) and Immm FSGSs, respectively. There are no data about the molecular structure of VN4; in the case of VN8, the molecular structure containing two dinitrogen groups and a η4-N4 planar group is most stable[117], i.e., the formula should be written as V(N2)2(η4-N4) (Figure 13a). For VN10, the most stable molecular structure is that similar to the structure of VN8 containing three dinitrogen groups and a planar η4-N4 unit; therefore, a more correct formula of this compound is V(N2)3(η4-N4) (Figure 13b)[117]. According to other data, the most stable structure of VN10 is V(η5-N5)2 similar to the ferrocene structure Fe(η5-C5H5)2, but unlike ferrocene, the point group of symmetry is D5h in this case[115]. However, this conclusion is in variance with the data of Ding et al.[117], who even does not mention V(η5-N5)2 among the possible molecular structures. The η4-N4 unit is also present in the only known vanadium HMN with an odd number of atoms, that is, VN9, the structure of which is depicted in Figure 13c.
Apart from TaN15 mentioned above, two more higher metal nitrides have been described for tantalum, namely, TaN4, TaN5 and Ta2N8[120-122]. The first one has the \( P2_{1}/m \) FSGS and incorporates a non-coplanar group of four nitrogen atoms[122]. For TaN5, the same authors found orthorhombic \( Fdd2 \) structure in which nitrogen atoms form branched chains, with each nitrogen atom having a tetragonal coordination. The nitrogen atoms incorporated in N3 groups shown in Figure 14 are bound to three Та atoms and one N atom; the N1, N2 and N5 atoms are bound to two Та atoms and two neighbouring N atoms; and N4 atoms are bound to one Ta atom and three N atoms. As regards Ta2N8 reported by Alkhaldi and Kroll[121], it was found to have \( P2_{1}/с \) FSGS similar to that of TaN4; however, in this structure, nitrogen atoms form a complex N810– anion. Hence, in the opinion of the authors, this compound should be considered exactly as Ta2N8 rather than TaN4. To conclude the discussion of higher nitrides of V, Nb and Ta, it is noteworthy that most publications devoted to these compounds include both theoretical and experimental data; furthermore, according to the authors of these studies, the theoretical and experimental results are in reasonably good agreement.
Higher nitrides of ns2(n – 1)d4 elements include only mononuclear compounds with even numbers of nitrogen atoms; they are considered in Refs [28, 109, 114, 123-127]. There is only one type of higher metal nitrides that are formed by all three elements of the indicated type, namely, MN6[123, 125, 127]. Two stable phases, \( Im3m \) and \( R3c \), existing at a pressure of ~ 100 GPa were found for CrN6 (Figure 15)[123]; analogous structures of MoN6 characterized by \( Im3m \) and \( R3m \) space groups exist at 50 GPa[125]. Only one stable phase with \( R3m \) FSGS was found for WN6; this structure is similar to that of MoN6 but arises at a higher pressure (165 GPa)[127]. There are two types of HMNs that are formed by two of these d-elements out of three, namely, MN4 and MN18. The MN4 type nitrides exist for M = Cr (see 28) and W[126]; compounds MN18 are formed for M = Mo and W[124]. Two crystal structures characterized by \( R3c \) and \( C2/m \) FSGS were predicted for CrN4, and one structure with \( Pbca \) FSGS was predicted for WN4. For experimentally obtained metal nitrides MN18, apart from the crystal structure (\( P3 \)), molecular structures were determined (both are d-metal hexaazides[124] with distorted octahedral coordination of azide nitrogen atoms bound to the metal). The metal nitrides found only for one metal of this group are CrN12 and WN10 reported by Mikhailov and Chachkov[109] and Bykov et al.[114], respectively. The authors[114] consider WN10 as WN8 · N2 the crystal structure of which (\( Immm \) FSGS) is composed of porous WN8 frameworks formed by W atoms, polydiazenediyl chains and dinitrogen units, which accommodate N2 molecules in the channels (Figure 16). The authors[114] believe that polydiazenediyl chains endow WN8 · N2 with fairly pronounced metallic properties (although in view of the specific features of the metallic bond, this explanation, frankly speaking, does not appear convincing). CrN12 has a molecular structure similar to that shown in Figure 16.
Higher nitrides of Group 7 (VIIB) elements, unlike other d-elements considered above, include derivatives of only two elements — Mn and Re[109, 126, 128-137]. There are no data on higher nitrides for Tc in the literature. The absence of experimental data on higher nitrides of this element is quite understandable, since stable isotopes of Tc are still unknown, while known isotopes are extremely hazardous due to their high radioactivity (it cannot be ruled out that, due to this circumstance, it was also inexpedient to consider them theoretically). Most of higher Mn and Re nitrides are objects of theoretical calculations; exceptions are provided by only MnN6, ReN8 and ReN10. It is characteristic that all higher nitrides of these elements described in the literature are mononuclear; the number of nitrogen atoms in them varies from 4 to 13 for Mn and from 3 to 10 for Re. The same stoichiometric composition is inherent in MN4, MN6 and MN8. Compounds of the first type are considered in Refs [126, 134, 135, 130-132]; the second type compounds are described in Refs [126, 131]. MnN4 has two stable and one metastable phases with \( P1 \), \( C2/m \) and \( P4/mmm \) FSGSs, respectively. The nitrogen-containing structural units in the first two structures include diatomic N2 molecules and S-shaped chains of nitrogen atoms, while the third structure contains N4 and N22 rings[131]. The Re compound of the same composition has a symmetry differing from that of MnN4, namely \( Pbca \), as well as from WN4[126, 134]. Zhao et al[135]. noted one more structure for ReN4, which has Cmmm FSGS. Apart from MnN4, three more higher nitrides of manganese were reported, namely MnN5 (\( P1 \)), MnN6 (\( C2/m \)) and MnN8 (\( P1 \))[131]. In all of these manganese compounds, there are strong covalent bonds between nitrogen atoms and weak Mn – N ionic interactions; a considerable contribution to stabilization of polymeric N-structures is made by the charge transfer between Mn and N atoms. It is noteworthy that all of the above-indicated structures are metallic phases[131]. Choi and Gillan[128] mentioned MnN6 as a precursor for the synthesis of manganese nitrides with lower nitrogen contents such as MnN and Mn3N2; however, no structural data for this HMN [except for the information that this is diazide Mn(N3)2] are given in the cited study. A \( R3m \) phase formed at P = 50 GPa was identified for ReN6; its structural fragment is shown in Figure 17[125]. Metal nitrides of type MN8 for d-elements of Group 7 (VIIB) were addressed by Niu et al.[131] and Bykov et al.[136] In the case of MnN8, a \( P1 \) phase was found[131]; in the case of ReN8, no similar phase was detected, but compounds ReN8 · xN2, similar to tungsten compounds described in another study[114] were described[136] (see Figure 16).
Higher manganese nitrides with an even greater nitrogen content, namely, MnN10, MnN12 and MnN13, were addressed in a number of theoretical publications[109, 129, 133]. The Mn(N5)2, i.e., manganese(II) bis(pentazolate), structure was proposed for MnN10[129]. Unlike other higher manganese nitrides, MnN12 and MnN13 contain macrocycles consisting of 12 nitrogen atoms; the only difference between them is that, apart from this macrocycle, MnN13 contains a nitride anion N3– axially oriented to the metal atom[133]. Note in this respect that the presence of the axial nitride anion in MnN13 has little influence on the molecular structure parameters compared to that of MnN12: in particular, in both HMNs, the N4 groups incorporated in the MnN4 chelate units are strictly coplanar, while these units themselves have virtually equal deviations from coplanarity (according to the results of DFT M06/TZVP calculations[109, 133], this is 54.8° in MnN12 and 56.0° in MnN13). Rhenium nitrides with these stoichiometriс compositions have not yet been reported in the literature; however, the existence of ReN10, the simplest compound ReN8 · xN2 with x = 1, was shown experimentally[136].
The range of known higher meal nitrides formed by elements of the next groups of the Periodic Table [Group 8 (VIIIB)] is generally wider than that for Group 7 (VIIB) elements; in all fairness, however, it should be noted that this diversity is mainly provided by higher nitrides of iron. These compounds are considered in Refs [82, 109, 113, 115, 128, 129, 133, 138-143]. Higher metal nitrides of other elements of this group, ruthenium and osmium, were described in only few studies[114, 126, 144]; most of the mentioned publications are theoretical. Currently, there is only one type of compounds known for each of these metals, namely, MN4 is known for M = Fe[143, 139-141], M = Ru[144] and M = Os[126]. A \( P1 \) phase has been found for FeN4[143, 139-141]) (Figure 18). However, a more detailed consideration using the CALYPSO method[142] revealed one more FeN4 phase with the same P1 group of symmetry, but differing from that described in the previous publications in the set of structural units formed by nitrogen atoms. In order to distinguish between these phases, Jiao et al.[142] designated them by \( P1 \)(a)-FeN4 and \( P1 \)(b)-FeN4; however, for some reason, they assigned the former designation to the structure mentioned in other studies[143, 139-141], and the latter designation was assigned to the structure that they described. Interestingly, a phase transition between these structures [\( P1 \)(b)-FeN4 to \( P1 \)(a)-FeN4] was predicted at P = 25 GPa; the scheme of the phase transition is depicted in Figure 18. A triclinic phase (\( P\bar{1} \) FSGS) stabilized at P = 37 GPa was also detected for RuN4. Like FeN4, it can in principle undergo phase transition at 62 GPa; however, unlike iron tetranitride, in this case, the triclinic \( P\bar{1} \) symmetry changes to tetragonal \( P4/mbm \) FSGS. The \( P\bar{1} \)-RuN4 structure contains a distorted RuN6 octahedron and an extended chain of nitrogen atoms (N∞), whereas P4/mbm-RuN4 consists of RuN8 rectangles and N2 units[144]. For OsN4 a structure with Pbca FSGS (Figure 19) was proposed[126], which is similar to the structures of WN4 and ReN4 HMNs with the same stoichiometry[126].
More nitrogen-rich higher iron nitrides include FeN5, FeN6, FeN8, FeN9, FeN10, FeN12, FeN13 and FeN15. The first one exists as four metastable phases with \( P4/mmm \) (a), \( P4/mmm \) (b), \( C2/m \) and \( P1 \) FSGSs (Figure 20)[142]. For FeN6, two phases were found (\( C2/m \) and \( P6_3 \)); for FeN8, there are four phases (\( Cc \), \( I4/m \), \( IPnnm \) and \( C2/m \)); and FeN10 has five phases (\( C2/m \), \( P1 \), \( Cm \), \( Immm \) and \( P2/m \)), only one of which is stable (\( C2/m \)-FeN6), whereas the other phases are metastable[142]. It is noteworthy in this regard that, although the \( C2/m \)-FeN6 phase exists over a broad range of pressures P (20 – 135 GPa), it is stable only at P = 35 – 55 GPa. The list of these phases and the pressure ranges in which they exist are presented in Figure 21. It is of interest that among the structures of FeN10 that are considered in this work, not a single one contains pentazolate groups N5. Therefore, attention is attracted by the results of publications[113, 115, 129] (by the way, they are purely theoretical, like the paper by Jiao et al.[142]) that predicted the possibility of existence of compound Fe(η5-N5)2 containing such groups. However, in each of the mentioned publications, this prediction was based on DFT data rather than on the results of crystal structure prediction methods; therefore, it is impossible to identify FSGS for this compound. As regards data on the structures of FeN6 and FeN8 given by Jiao et al.[142], they are quite consistent with the results reported for these compounds by other authors[138, 141, 143]. Among higher iron nitrides with an even number of nitrogen atoms, the most nitrogen-rich one is FeN12[109], the structure of which is similar to the structures of other nitrides MN12 described in the same publication. Among higher iron nitrides with odd numbers of nitrogen atoms, apart from FeN5, recent publications mention FeN9[128], which is iron(III) triazide Fe(N3)3, and FeN13[133], which is structurally similar to the manganese compound with the same nitrogen to metal stoichiometric ratio and was also studied by Mikhailov and Chachkov[133]. Mention should also be made of the compound with the highest nitrogen content, FeN15, which is tris(pentazolato)iron(III) Fe(η5-N5)3, according to the data of Chen et al[82].
To conclude the discussion of higher nitrides of this group of d-metals, note the only polynuclear compound among all Fe, Ru and Os higher nitrides, namely, Os5N34, reported by Bykov et al[114]. A fragment of its crystal structure is shown in Figure 22. Actually, this is nothing other than Os5N28 · 3N2; like WN8 · N2 and ReN8 · N2, it also crystallizes in Immm FSGS and contains porous Os5N28 frameworks formed by Os atoms, polydiazenediyl chains and dinitrogen units, with N2 molecules being located in the channels.
Group 9 (VIIIB) elements with electron configuration ns2(n – 1)d7, namely, Co, Rh and Ir, are quite comparable with Group 3 (IIIB) elements, which were discussed in the beginning of Section 2.3, both in the diversity of HMNs they form and in the number of publications addressing them. These elements are also typically form mononuclear metal nitrides (most often, with even numbers of nitrogen atoms), although polynuclear metal nitrides are also known for each of these elements. These compounds are mentioned in one or another aspect in quite a few publications[109, 113, 115, 129, 145-152]; the number of nitrogen atoms per metal atom in them varies from 3 (in IrN3) to 16 (in RhN16). In this regard, it is worth noting that higher k : m ratio was observed only in MN20 (M = Ti, Zr, Hf) and WN18. For Group 9 (VIIIB) elements, there is only one type of mononuclear HMNs that is formed by each of the elements, namely, MN4. There are also types of nitrides formed by two elements, in particular MN15 (M = Rh, Ir) and MN8 , MN10 (M = Co, Rh).
It is of interest that d-elements of this group form one more type of higher metal nitrides, which are characteristic of Co, Rh and Ir; these are binuclear compounds M2N20 described by Tang et al[145, 147, 151]. It is noteworthy that no other such examples have yet been found among polynuclear HMNs in theoretical calculations or experimentally.
The simplest higher nitrides of Group 9 (VIIIB) elements, namely MN4, have been considered in Refs [146, 148, 152], respectively. It is noteworthy that higher Co and Ir nitrides were studied only theoretically, whereas higher nitrides of rhodium were, most often, detected experimentally. CoN4 forms a stable \( P\bar{1} \) phase in which strong covalent N–N interactions are present; moreover, according to the authors, this phase is metallic[146]. For polynitride RhN4 reported by Brathwaite et al.[148], no data on the crystal structure are available; it is only known to contain two dinitrogen groups. Therefore, it should be noted that the same groups of nitrogen atoms, only in larger numbers, are also present in more complex nitrides, RhN6, RhN8, RhN10, RhN12, RhN14 and RhN16, which were detected experimentally[148]. However, structural data on these HMNs given in the cited publication are scarce. For example, it is indicated that RhN10 and RhN12 have the geometry of a tetragonal pyramid with \( C_{4v} \) symmetry and a tetragonal bipyramid with \( D_{4h} \) symmetry with dinitrogen N2 groups at the vertices, respectively. The third representative of type MN4, namely IrN4, was investigated in more detail by Du et al.[152], who predicted the existence of stable IrN4 (\( R3с \) FSGS) and IrN7 ((\( P2_1 \) FSGS) in the ranges P = 40 – 100 and 30 – 50 GPa, respectively, using the CALYPSO method. In both IrN4 and IrN7, the metal atoms have C.N. = 6 and are located at the geometric centre of the coordination polyhedron typical of this coordination number, that is, octahedron, the vertices of which are occupied by nitrogen atoms. In IrN4, tetrahedral nitrogen atom groups are coordinated to iridium (Figure 23a), while in IrN7, iridium coordinates four dinitrogen groups in the equatorial plane of the octahedron and two pentazolate groups (Figure 23b) in the axial positions of the octahedron[152]. In the case of M = Co, heptanitride has not yet been detected, and CoN8 proves to be the second simplest after CoN4 among higher nitrides described in recent years[146] [although, a simpler cobalt compound with nitrogen should also exist, in particular, cobalt(II) diazide CoN6, which however, was not mentioned in recent publications]. According to Liu et al.[146], CoN8 has a layered structure formed by nitrogen atom; the phase formed by this compound is metastable and has \( P\bar{3}1c \) FSGS. The same authors found another higher nitride of this element, CoN10, in which nitrogen atoms form a band structure. Like \( P\bar{3}1c \)-CoN8, it is metastable and has the same P1 – FSGS as CoN4. Mikhailov and Chachkov[109] theoretically described the higher cobalt nitride CoN12, which is distinguished (at least, at the moment) among known higher nitrides of this element by the maximum content of nitrogen; however, only the molecular structure, similar to that depicted in Figure 11, was determined for this compound. In the case of Rh and Ir, HMNs with even greater number of nitrogen atoms per metal atom (MN15) are known[113]. Both compounds are tris(pentazolates) of the corresponding elements, i.e., they can be described by the formula M(N5)3, and each of them forms two stable phases with \( P2_{1}/m \) and \( P1 \) FSGS, the first of which exists at P = 0 – 50 GPa and the second exists at P > 50 GPa (Figure 24).
The molecular structures of higher binuclear metal nitrides M2N20 (M = Co, Rh, Ir) were studied by DFT with the B3LYP functional[145, 147, 151]. Tang et al.[145] identified five molecular structures for each of the three compounds; the \( D_{4h} \) structure proved to be most stable for each M (Figure 25). A notable feature is the presence of metal – metal bonds, which is not characteristic of metal nitrides, in general, and higher nitrides, in particular. The results of this study were confirmed in other publications[147, 151]. Therefore, it should be noted, as mentioned above, that Co and Rh form compounds MN10 in which the k : m ratio is the same as in compounds shown in Figure 25; however, their structures differ appreciably from the structures of M2N20; thus, the type of isomerism called coordination polymerism is involved in this case. Cui et al.[149] mentioned the trinuclear nitride Rh3N9, which also contains metal – metal bonds in the opinion of the authors.
Unlike Group 8 and 9 elements, HMNs of Group 10 (VIIIB) elements are mentioned in the literature only occasionally[109, 113, 128, 129, 153]. Virtually all publications addressing these compounds, except a paper by Choi et al.[128], are purely theoretical. In the case of Ni, higher nitrides NiN6, NiN10 and NiN12 were found, while for Pd, only PdN10 is known and for Pt, PtN4 and PtN5 have been reported. These two platinum compounds are the simplest higher nitrides of the above elements; they are considered in a recent publication[153]. According to the reported data, both compounds are stabilized at a relatively low pressure P ~ 50 GPa, and both correspond to \( P\bar{1} \) FSGS. In the order of increasing k : m ratio, the next compound is NiN6, which is nickel(II) diazide[128]. There is only one type of HMN formed by different elements of Group 10, particularly MN10 (M = Ni, Pd)[113]. Both compounds are characterized by \( P2_{1}/c \) FSGS and represent bis(pentazolates) of the corresponding metals M(N5)2; a fragment of the crystal structure of these phase is shown in Figure 26. The highest k : m ratio for higher nitrides of these elements was noted in the compound NiN12[109]; its molecular structure is similar to the structures of analogous compounds MN12 formed by other 3d-elements and also considered in this publication.
The inventory of HMNs formed by Group 11 (IB) d-elements is somewhat more abundant, although these compounds are currently known only for two of these elements, namely, for Cu and Ag. Higher nitrides of these elements were considered in a number of publications[109, 113, 154-160], each being a purely theoretical study. The simplest HMNs are CuN3 and AgN3[156, 158], which are nothing other than the long known Cu(I) and Ag(I) azides with rather simple molecular structures and, hence, they do not need to be considered in detail here. It is only noteworthy that, apart from the previously known Ibam phase, two other phases (\( I4/mcm} \) and \( P\bar{1} \)) existing at P = 4.7 – 24 GPa and P > 24 GPa, respectively, were predicted[158] by the CALYPSO method. In addition to the above-indicated monoazides, two more types of HMNs formed by both of these d-metals were found, namely, CuN5 and CuN6 (and, correspondingly, AgN5 and AgN6). The pentanitride CuN5 is Cu(I) pentazolate. The CALYPSO method identified one phase (\( P2_{1}/m \) FSGS) for this compound; in its crystal structure, each metal atom is bound to two N5 groups, while each such group is bound to two metal atoms[154]. In a later study[155], three more phases were detected for this compound, namely, \( P2_{1}2_{1}2_{1} \)-CuN5, \( P2_{1}/c \)-CuN5 and \( P4_{1} \)-CuN5; however, it was noted that, as opposed to the data of Li et al.[154], the \( P2_{1}/m \)-CuN5 structure found by Zhao and co-workers[155] is less energetically favourable than any of the above structures, while \( P2_{1}2_{1}2_{1} \)-CuN5 is most favourable (the total energies of \( P2_{1}/c \)-CuN5, \( P4_{1} \)-CuN5 and \( P2_{1}/m \)-CuN5 exceed the total energy of \( P2_{1}2_{1}2_{1} \)-CuN5 by 0.02578, 0.06372 and 0.42453 eV, respectively[155]). Nevertheless, each of these structures contains pentazolate groups, and the main difference between them is that the Cu atoms in the stable \( P2_{1}2_{1}2_{1} \)-CuN5 (Figure 27a) and \( P2_{1}/с \)-CuN5 (Figure 27b) phases have C.N. = 4 with a tetrahedral coordination of nitrogen atoms to copper, whereas in the metastable \( P4_{1} \)-CuN5 and \( P2_{1}/m \)-CuN5 phases, the copper C.N. is 3 (trigonal coordination) and 2 (linear coordination), respectively[155]. It was noted in both publications that all of these compounds are formed in the pressure range from 0 to 100 GPa; however, particular pressure ranges for each compound were not indicated in the publications. AgN5, similar to CuN5 in the composition, also contains pentazolate groups (like CuN5), but exists as a \( P1 \) phase, which has not yet been found for copper(I) pentazolate in either theoretical or experimental works[113]. The existence of silver(I) pentazolate was also mentioned by Sun et al.[156] and Williams et al.[157], but no data on its crystal structure were reported. In this regard, attention is attracted by a study of Liu et al.[160], who also considered the AgN5 phase with \( P1 \) FSGS and noted the presence of infinite bands of nitrogen atoms, but did not mention the presence of N5 groups.
The studies mentioned above report also higher nitrides MN6 of these elements. Despite their identical stoichiometric composition, they have markedly different structures: CuN6 is copper(II) diazide[154], while AgN6 contains pentazolate and dinitrogen groups[157]. This difference should be attributed, at least, to the fact that the oxidation state of +2 [which is characteristic of Cu and occurs in Cu(N3)2] is unusual for Ag. Liu et al.[159] characterized three more higher silver nitrides, AgN4, AgN7 and AgN8; according to the results of CALYPSO calculations, there are metastable phases with \( P\bar{1} \) FSGS for AgN4, AgN7 and AgN8 and a phase with \( P1 \) FSGS for AgN7; the three first-mentioned phases appear at P = 150 GPa, whereas the fourth one exists at a considerably lower pressure (50 GPa). The \( P\bar{1} \)-AgN4 contains N6 rings connected by dinitrogen N2 groups (Figure 28a); \( P1 \)-AgN7 is formed by pentazolate N5 units and dinitrogen N2 groups (Figure 28b); \( P\bar{1} \)-AgN7 is composed of N7 rings connected by N2 groups (Figure 28c); and \( P\bar{1} \)-AgN8 is formed by 18-membered nitrogen macrocycles connected by the same N2 groups. It is of interest that a similar 18-membered nitrogen ring was found 100 in yttrium hexanitride YN6, although the stoichiometric compositions of compounds containing this ring differ from each other. The paper also mentions the compound AgN10, but no information of its molecular or crystal structure is given.
A study mentioned above[109] describes the compound CuN12, the molecular structure of which determined by various versions of the DFT method, resembles the structures of compounds MN12 formed by other d-elements, but is distinguished by the smallest non-coplanarity of the MN4 group and simultaneously the highest non-coplanarity of the 12-membered nitrogen macrocycle.
To conclude the discussion of the specific features of higher metal nitrides of this group of d-elements, it should be noted that there is still no published data on the higher nitrides of gold, the heaviest d-element of this group; however, there are no conceptual theoretical objections to the possibility of their existence either (at least, for azides or pentazolates).
Finally, Group 12 (IIB) d-elements, like their neighbours in the Periodic Table, do not exhibit a particular variety of stoichiometric compositions of metal nitrides (in particular, HMNs) they form[129, 161-167]. All studies addressing these compounds are theoretical and are devoted to calculations of crystal structure parameters. Five types of HMNs formed by two elements out of three were found for elements of this group: MN3 (Zn, Hg) and MN4, MN5, MN6 and MN10 (Zn, Cd). Two stable phases corresponding to the same \( P1 \) space group were found[165] for the simplest compound, ZnN3: one phase contains separate acyclic N6 groups and exists in the pressure range of 54 – 72 GPa, while in the other phase existing above 72 GPa, these groups have transformed into infinite chains; a semiconductor-to-metal phase transition was also predicted. Apart from the two stable phases, a metastable phase (\( Cmmm \)) formed at a pressure of 100 GPa was revealed for this compound[162]. For a mercury compound analogous to ZnN3 (HgN3), Guo et al.[167] proposed \( P2_{1}/c \) (Figure 29a), \( I4/mcm \) (Figure 29b), \( C2/m \) (Figure 29c) and \( P2_{1}/m \) (Figure 29d) phases and two \( P\bar{1} \) phases [designated by the authors as \( P\bar{1} \) (Figure 29e) and \( P\bar{1}\_I \)(Figure 29f )][167]. It is worth noting that, although this compound was interpreted[167] as mercury azide, the azide N3 groups are actually present only in \( P2_{1}/c \)-HgN3 and \( I4/mcm \)-HgN3, while other structures contain either simpler N2 groups (\( C2/m \)-HgN3) or, conversely, more complex groups consisting of five, six or eight nitrogen atoms; therefore, it is inappropriate to consider four of these six phases as mercury azides.
Group 12 (IIB) d-element tetranitrides, that is ZnN4 and CdN4, were described a few studies[162, 163, 166]. Three stable phases were identified for ZnN4, characterized by \( P\bar{1} \), \( C2/c \) and Ibam FSGSs and existing at pressures of 20 – 98 GPa, >55 GPa and >98 GPa, respectively[162]; the structure of each phase contains zigzag-like N4 groups. For CdN4, Niu et al.[166] found two stable phases with Cmmm and \( I\bar{4}2d \) FSGSs, in both of which nitrogen atoms form non-linear chains consisting of eight atoms. The \( Cmmm \)-CdN4 exists in the pressure range P = 21 – 117 GPa and \( I\bar{4}2d \)-CdN4 is formed at P = 117 – 150 GPa.
The publications mentioned above[165, 166] also describe type MN5 and MN6 nitrides. According to these studies, ZnN5 forms one stable phase (\( Cmm2 \)), in which the nitrogen atoms form three-dimensional network structures[165], and metastable phases with \( P1 \), \( P\bar{1} \), \( C2/c \) and \( P2_{1}/m \) space groups arising at P = 50 GPa[162]. Unlike ZnN5, the Cd compound with the same stoichiometry forms a stable phase with \( C2/c \) FSGS in which the nitrogen atoms form 14-membered zigzag – antizigzag chains (Figure 30a). This compound is formed at very high pressures (above 120 GPa). A structure with \( Pcc2 \) space group in which the nitrogen atoms form two-dimensional network layers was identified for ZnN6; the CdN6 structure (\( C2/m \) semiconductor-to-metal phase transition) has a unique structural unit composed of 14 nitrogen atoms (Figure 30b)[166]. The most nitrogen-rich HMNs of these elements, MN10, are bis(pentazolates) of the corresponding metals[161]. Unfortunately, no information regarding FSGSs of these compounds can be found in the publications.
Data on the stoichiometric composition of higher polynitrides of d-elements are summarized in Table 3.
2.4. Homometallic higher metal nitrides of f-elements
Currently, compounds of this type have been reported only for three 4f- and two 5f-elements, particularly for Ce, Gd and Er, Th and U; furthermore, all publications that are devoted to 4f-element HMNs refer to the last five-year period. Like for higher s-, p- and d-element nitrides, in this case, too, theoretical studies clearly predominate (Refs [168-173]); experimental data are given only in two publications[174, 175]. Six higher nitrides were identified for cerium, namely, CeN4, CeN5, CeN6, CeN8, CeN10 and CeN15[168-171]; three nitrides were found for gadolinium (GdN3, GdN4 and GdN6)[172]; there are two nitrides for erbium (ErN4 and ErN6)[173]; only one nitride is known for thorium (ThN6)[105] and twelve nitrides were described for uranium (UN4 to UN14 and UN16)[174, 175].
The simplest compounds are MN4, which were reported for Ce[168], Gd[172] and Er[173]. The first-mentioned HMN forms a I41/a phase in which nitrogen atoms are joined into helical N4 chains, the second one forms a \( P1 \) phase and the third one forms a phase with \( P\bar{1} \) FSGS group in which nitrogen atoms form armchair type structures. For these three 4f-elements, higher metal nitrides MN6 were also described. According to reported data[170-173], CeN6 and ErN6 form phases with \( P\bar{1} \) FSGS, while the GdN6 forms a \( P1 \) phase. It is of interest that structural moiety of 14 nitrogen atoms, similar to that shown in Figure 29b, is formed in CeN6. One more HMN series is known for cerium, unlike both gadolinium and erbium and unlike other lanthanides. As regards structural diversity, CeN5 appears to be the most interesting: four stable phases with \( C2 \), \( P\bar{1} \), \( P2/c \) and \( P2_{1}/m \) FSGSs were identified for it[169]. These phases were predicted to appear in the pressure ranges of 0 – 20, 20 – 50, 50 – 100 and above 100 GPa, respectively. It is important that almost all these phase, except for only \( P2/c \), can be, to quote the authors, quenched (i.e., stabilized) to exist under ambient conditions. Three more higher nitrides of this f-element, namely, CeN8, CeN10 and CeN15, have been described[168] using the MAGUS method: the first of these compounds forms two phases with \( P\bar{1} \) and \( P\bar{3} \) – space groups; the second compound containing a pentazolate anion forms \( I4/m \) phase; and the third one, which is also cerium pentazolate Ce(N5)3, forms two phases (\( C2 \) and \( P2_{1}2_{1}2 \)). It is noteworthy that CeN10 is a ferromagnet, whereas CeN15 exhibits antiferromagnetism[168].
It is known that all 5f-elements are radioactive and not a single stable isotope has been found for any of them so far; for this reason alone, it is very difficult to carry out experimental studies of HMNs of these elements, and only theoretical works dealing with these nitrides are available[95, 174, 175]. Straka[105] performed the molecular structure calculation for ThN6, which, like the higher metal nitrides MN6 of Group 4 (IVB) elements considered in the same publication, is a π-complex containing a planar N6 ring coordinated to the Th atom by all six nitrogen atoms. This study was performed in the early 21st century and since then, no data about this compound or other higher binary HMNs containing only Th and N atoms appeared in the literature. A much greater diversity of higher metal nitrides was observed for the third 5f-element in the ascending order of numbers, uranium, for which the complete set of stoichiometric compositions of UNk in the range k = 4 – 16 was reported[174]. Each compound is either UN(N2)l (in the case of UNk with odd k) or U(N)2(N2)l (for UNk with even k) and contains one or two U≡N triple bonds, respectively, and dinitrogen N2 groups in the molecule. Examples of such molecular structures are shown in Figure 31. In this respect, attention is drawn by the fact that in all HMNs with even numbers of nitrogen atoms, those N atoms that are bound to uranium are located in one plane and occupy the vertices of the equatorial planes of a regular trigonal (in UN8), square (in UN10), pentagonal (in UN12) or hexagonal (in UN14) bipyramid, whereas in the case of HMNs with odd numbers of nitrogen atoms starting with UN9, this is not observed (see Figure 30). It is also noteworthy that in higher metal nitrides UN4 and UN8, the bond angles formed by uranium atoms with nitride N atoms, N – U – N, are markedly (by 10° and more) differ from 180°[174]. In a later study, Marks et al.[175] considered single- charged molecular ions of higher uranium nitrides with the same even numbers of nitrogen atoms in the structural units as used in the earlier study[174], but, unlike them, containing only N2 groups. These compounds were detected experimentally using IR spectroscopy and mass spectrometry, but their molecular structures, like those reported by Andrews et al.[174], were calculated theoretically using the DFT method. No data on the possible phases of these compounds and their FSGSs have yet been reported in the literature.
Data on the stoichiometric composition of higher polynitrides of f-elements are summarized in Table 4.
As can be seen from the foregoing, the array of data on homometallic higher metal nitrides is quite extensive. According to the author’s estimate, the above publications describe the molecular structures (calculated theoretically or found experimentally for the gas phase) and crystal structures (calculated theoretically or found experimentally for the solid phase) for a total of 356 such compounds (97 molecular and 259 crystalline compounds), among which 94 are s-element nitrides, 40 are p-element nitrides, 196 are d-element nitrides and 26 are f-element nitrides. The subdivision of the 259 crystal structures of the compounds considered in the review in terms of the structural motifs [molecular (0D), chain (1D), layered (2D) or framework (3D) motif] demonstrated that 76 compounds are chain-like, 97 are layered and 86 are framework structures. Meanwhile, no crystal structures that could be classified as molecular (that is, those in which MmNk molecules rather than N atoms or structural units of nitrogen atoms are located in the points of the crystal lattice) were found among these compounds. The distribution of these structural motifs among higher metal nitrides of s-, p-, d- and f-elements is summarized in Table 5.
The characteristic nitrogen atom groups present in these higher metal nitrides are depicted in Figure 32. Notably, among them there are no molecular structures typical of higher polynitrogens such as N4 as a regular tetrahedron, N6 as a prism, N8 as a cube and many other structures that were considered in quite a few studies devoted to polynitrogens (see, in particular, reviews[12-14]). Meanwhile, among the groups shown in Figure 32, there are groups that have not yet been found in polynitrogens [e.g., zigzag-like N8 (Figure 32n), bicyclic N14 (Figure 32s) and macrocyclic N18 (Figure 32t)]. The structural group most abundant in the compounds in question is the N5 ring (Figure 32g) (most often, planar); groups i, m and o are also encountered rather frequently. No definite correlation has yet been identified between the gross formula of the higher metal nitride and the presence of atomic groups out of those shown in Figure 32, although it can be noticed that homometallic metal nitrides with 10, 15 and 20 nitrogen atoms often contain five-membered rings (Figure 32g), while metal nitrides in which the number of nitrogen atoms is 6 or 8 often incorporate hexa- or octa-atomic structural groups (Figure 32i – k and Figure 32m – o, respectively) (this, however, appears quite natural). Among molecular structural motifs (0D), the predominant ones are those that have relatively low symmetry and correspond, as a rule, to \( C_s \), \( C_{2v} \) or even \( C_1 \) types according to the Schoenflies notation; the number of molecular structures characterized by these symbols is more than 50% (55 out of 97). The number of structures corresponding to \( D \) and, the more so, \( T \) and \( O \) types according to the Schoenflies notation is moderate (14); thus, it can be concluded that, in general, highly symmetric molecular structures are very untypical of these compounds.
3. Heterometallic higher metal nitrides
Nitrides containing atoms of two different metals have been known for quite a long time and are rather numerous; reviews mentioning at least several dozens of such compounds were published back in the late 20th century[176, 177]. Quite a few studies devoted to these nitrides also appeared in the 21st century; data on some of them can be found in a relatively recent review[178]. However, strictly speaking, it must be admitted with regret that among the compounds mentioned in these studies in one way or another, there are actually no examples that could be classified as particularly HMNs in terms of the systematics proposed in the Introduction (i.e., compounds in the structural units of which the ratio of the number of nitrogen atoms to the total number of metal atoms would be greater than 3). Therefore, this Section covers only those heteronuclear metal nitrides in the structural units of which the total number of metal atoms is at least not greater than the number of nitrogen atoms and the number of nitrogen atoms is not less than three. However, even with this expanded meaning of the term ‘higher metal nitrides’, the number of publications addressing these compounds is modest[179-214]. Therefore, it should be stated that the studies concerning particularly such metal nitrides obviously need to be further developed.
3.1. Heterometallic metal nitrides of type MM'N3 with the perovskite structure
Judging by the number of publications, the greatest attention of researchers is attracted by compounds generally described as MM'N3 , which contain atoms of two different metals M and M' and three nitride anions N3–. These compounds are considered in a number of publications[179-194], which are mainly theoretical; only few papers present some experimental results[179, 189-192]. As a rule, the compounds have a perovskite CaTiO3 or a similar crystal structure. Two main types of such HMNs have been reported in the literature: in one of them, the oxidation states of M and M' are +4 and +5, while in the other, the oxidation states are +3 and +6, respectively. Theoretically, there are two more options for MM'N3[180], particularly, the oxidation states of M and M' may be +2 and +7, or +1 and +8, but there are no reliable data concerning the possibility of their existence (although it cannot be ruled out that the option with +2 and +7 oxidation states for M and M' is actually implemented in HMNs CaTcN3, SrTcN3, CaReN3, SrReN3 and BaReN3, which were considered by Sarmiento – Pérez et al.[185]).
It is of interest that trinitride ThTaN3 was one of the first compounds of this type to be reported in the literature[179-183]. In the earliest study[179], this compound was obtained experimentally and was shown to crystallize in the \( Pm3m \) space group. In a later study[181], two more possible phases (\( Pnma \) and \( Cmcm \)) were identified for this compound by quantum chemical simulation; according to the authors, the first mentioned phase is formed at P = 260 GPa, the second one appears at 262 GPa, and the third one is generated at 268 GPa. Two of these structures, namely the cubic \( Pm3m \) and orthorhombic \( Pnma \) phases, were also addressed in another study[182]. This compound can be considered unique, because, first, it is the only known higher metal nitride of type MM'N3 that contains a 5f-element atom and, second, it is one of the few compounds of this type in which atoms M and M' are in +4 and +5 oxidation states. In this respect, it should be noted that, apart from ThTaN3, only two other such nitrides are known, namely, CeTaN3 and CeNbN3, reported recently by Ha et al[184]. Both these heteronuclear HMNs, like ThTaN3, were noted to form Pnma phase. However, Ha et al.[184] did not mention the other two phases (\( Pm\bar{3}m \) and \( Cmcm \)) that were identified for this compound in earlier studies[179, 181].
A much higher diversity of perovskite-like metal nitrides of type MM'N3 is characteristic of compounds in which the oxidation states of M and M' are +3 and +6[185-194]. As a rule, the metals M present in these compounds are 4f-elements, while M' are Group 6 and 7 elements, first of all, Mo, W and Re, but there is also an example of metal nitride MM’N3 in which M' is represented by group 8 (VIIIB) element, namely, Os in LaOsN3[185]. Fragments of the crystal structure of some heterometallic metal nitrides with \( C2/c \) and \( C2/m \) FSGSs considered in this study are shown in Figure 33a,b, respectively.
A detailed DFT study[186] of LaWN3 revealed nine phases, with \( R3c \), \( Pna2_1 \), \( R3m \), \( Pnma \), \( R\bar{3}c \), \( P4/mbm \), \( I4/mmm \), \( I4/mcm \) and \( Pm\bar{3}m \) FSGSs, the energy of which increases in the indicated order; apparently, the first of them can be considered to be stable, while the other ones have much higher total energy and can be classified as metastable. The fact that \( R3c \)-LaWN3 is the most stable phase for this polynitride was noted in a later theoretical study[187], in which, apart from this compound, LnWN3 and LnReN3 (where Ln is a 4f-element) were investigated by DFT. These compounds were also addressed in other papers[188, 190, 193]. It is characteristic that a stable phase with the same FSGS was also noted for two other LnWN3, namely, EuWN3 and YbWN3 (Table 6), in which the 4f-elements have 7 and 14 electrons in the f-orbitals (fully and half filled electron shells); for other LnWN3 (except for CeWN3), a stable phase with \( C2/c \) FSGS is implemented. The same feature of Eu and Yb compounds is also manifested for metal nitrides LnReN3, although the type of structure and FSGS and the type of electronic conductivity for each of them differ considerably from those for LnWN3 (see Table 6); the only exception here is Ce for which the indicated characteristics of these HMNs coincide. However, it is worthy of note in this respect that a phase with \( I\bar{1} \) FSGS was found experimentally for LaReN3 by Kloß et al.[191], who, however, did not mention the \( Pnma \) phase, which was identified for this compound by Flores-Livas et al[187].
Tungsten and rhenium are the chemical elements most often encountered as M'; other studied HMNs contain Tc (\( C2/c \)-YTcN3, \( C2/c \)-LaTcN3, \( P2_{1}/m \)-CaTcN3 and \( P2_{1}/m \)-SrTcN3), Mo (\( C2/c \)-YMoN3, \( C2/c \)-LaMoN3) and Cr (\( C2/c \)-LaCrN3)[185]. A polynitride MM'N3 with M' = Mo, namely, CeMoN3 with \( Pmc21 \) FSGS has been described[182, 184], together with an analogous W compound. Their spatial structures are shown in Figure 34.
As a conclusion of this Section, it is noteworthy that Adeleke et al.[185] described SrBaN3, which is similar in the stoichiometric composition to the compounds considered above, but differs by the fact that it contains only two Group 2 (IIA) elements, each in the +2 oxidation state; if the nitrogen oxidation state is taken to be –3, this compound does not formally fit into the traditional views on the valence capabilities of these metal atoms. Two structures were identified for this compound: cubic (\( P\bar{4}3m \) FSGS) and tetragonal (\( P4/nmm \) FSGS); the former, which is energetically more favourable, arises at P = 4.92 GPa, while the latter is formed at P = 7.23 GPa. Fragments of these structures are depicted in Figure 35. As can be seen, one phase contains isolated chains of four nitrogen atoms as structural units, while the other one includes groups of seven nitrogen atoms. Thus, none of these phases contains nitride anions. In addition, there are several other compounds the composition of which, like that of SrBaN3, also does not formally fit into the traditional views on the valence capabilities of the involved metals, namely, CaMoN3, CaWN3, SrWN3, BaWN3, LaTaN3, LaVN3 and LaCoN3[185].
Data on the stoichiometric composition of higher metal nitrides MM'N3 of s-, p-, d- and f-elements with a perovskite-like structure are summarized in Table 7. As can be seen, classification in terms of the classes of chemical elements present in the nitrides as metal pairs (MM') includes only four combinations out of the ten theoretically possible ones: (ss), (sd), (dd) and (fd); the numbers of characterized HMNs are quite different and increase on going from the first to the fourth combination.
3.2. Non-perovskite heterometallic polynitrides
The group of heterometallic nitrides that is next in terms of the research interest (and that can be referred to as ‘higher nitrides’, according to the above-described approach) includes compounds with the composition M2M'N3, which were addressed in a number of studies[196-207]. It is noteworthy that, unlike the homo- and heterometallic higher nitrides considered above, HMNs of this type were mainly obtained experimentally and were characterized using various physicochemical methods (spectral, magnetic, etc.); only one study can be considered as purely theoretical[196]. The polynitrides Th2CrN3, Th2MnN3, U2CrN3 and U2MnN3 were the first to be obtained experimentally. According to X-ray diffraction data, each of them has the Immm FSGS[196]. In a later publication[197], the synthesis of Ba2NbN3 with a similar stoichiometry was reported. According to X-ray diffraction data, the compound had \( C2/c \) FSGS. Later, the list of the studied metal nitrides M2M'N3 was supplemented by several more representatives, namely, Ce2CrN3 and U2CrN3 (both Immm)[198], Sr2VN3 (\( C2/c \)) and Ba2VN3 (\( Cmca \))[199], Ce2MnN3 (\( Immm \))[200, 201], Ca2VN3 (\( C2/c \))[202], Sr2TaN3 (\( C2/c \))[203], La2GaN3 (\( C2/c \))[204] and Ce2CrN3 (\( Immm \))[205]. As can be understood from the above, the most common type of symmetry is Immm; an example of such structure is shown in Figure 36.
Most of these compounds have been prepared experimentally at different times and investigated using X-ray diffraction analysis. Among the relevant publications, note a paper by Bowman and Gregory[203] devoted to the synthesis and characterization of Sr2TaN3 including SEM imaging of its polycrystals (Figure 37). This is a rare example among the whole array of publications dealing with both homo- and heterometallic higher nitrides.
For the M and M' elements present in the metal nitrides M2M'N3, provided that they contain particularly three nitride anions, like for MM'N3, quite a few combinations of oxidation states are possible, namely, (+3, +4, +2), (+2, +5, +2), (+3, +3, +3), (+2, +4, +3), (+1, +5, +3), (+2, +3, +4), (+1, +4, +4), (+2, +2, +5), (+1, +3, +5) and (+1, +2, +6) (the first two digits correspond to the M atoms and the third one refers to the M' atom. The combinations in which the oxidation states are typed in bold appear unlikely due to intramolecular redox reactions accompanied by a decrease in the high oxidation state of one M atom and increase in the oxidation state of the other atom of the same type). Some of the above-mentioned metal nitrides can be easily assigned to a particular type of those listed above: indeed, Ba2VN3 and Sr2TaN3 correspond to (+2, +2, +5), and La2GaN3 and Ce2CrN3 correspond to (+3, +3, +3). However, in most cases, assignment of a metal nitride to some of the above-mentioned variants is faced with certain difficulties. This is manifested especially clearly when both M and M' are able to exist in different oxidation states. However, this issue receives little attention in the literature devoted to these compounds[196-207]. Among the few exceptions are publications by Niewa et al.[200, 201], who considered three hypothetical options for Ce2MnN3, namely, (CeIV)2MnIN3, CeIIICeIVMnIIN3 and (CeIII)2MnIIIN3, i.e., (+4, +4, +1), (+3, +4, +2) and (+3, +3, +3), respectively. The first option seems a priori doubtful, since CeIV is a very strong oxidant, while MnI is a very strong reducing agent; therefore, they can hardly exist simultaneously in the same compound; indeed, judging by X-ray absorption spectroscopy data[201], the most probable formula is CeIIICeIVMnIIN3. The identification of the appropriate options for Th2CrN3, Th2MnN3, U2CrN3 and U2MnN3 appears even more problematic[196]. In view of the fact that these compounds crystallize in the same Immm FSGS as Ce2CrN3, in which the (+3, +3, +3) combination of oxidation states seems to cause no doubt, it is possible to assume something like that for these compounds, too. Unfortunately, a recent study[207] devoted to U2CrN3 does not address this issue in any way.
Higher heterometallic nitrides with stoichiometric compositions other than MM’N3 and M2M’N3 mentioned above are also occasionally mentioned in the literarure[208-214]. Among them are a few compounds with the general formula M3M'2N6, where M = La, Ce, Pr, M' = Nb, Ta[208], La3V2N6[209], La3Cr2N6[210], all having \( I4/mmm \) FSGS. The presence of nitride anions was also postulated for these compounds. The same gross formula can be assigned to the heteronuclear metal nitride Li2Ca3N6 with \( Pmma \) FSGS[211], which contains diazenide N22– rather than nitride anions; therefore, its formula was given as Li2Ca3(N2)3. A structural fragment of this compound is shown in Figure 38. All these compounds were obtained experimentally and characterized by appropriate physicochemical methods. Apart from these metal nitrides, other reported compounds include AlBiN4[212], LiBe2N6, Li2Be2N6 (Ref. [213]) and LiMgN4[214], which are formed in Be2N6 and MgN4 thin films upon lithium doping. The data on this issue, however, look quite scattered and obviously need to be supplemented, especially since they were again obtained by theoretical calculations.
Data on the stoichiometric composition of non-perovskite higher metal nitrides of s-, p-, d- and f-elements are summarized in Table 8. Here, one can found seven out of the ten theoretically possible MM' combinations, in terms of classification according to types of chemical elements; apart from the four combinations mentioned in Table 7, there are three options involving p-elements: (sp), (pp) and (pd). As in the case of perovskite HMNs, the greatest number of non-perovskite nitrides belong to the (fd) type.
To summarize this Section, we would like to note that the cited publications include data on 96 crystal structures, 75 of which are perovskite metal nitrides, which by definition are characterized by a 3D structural motif; the other 21 nitrides are non-perovskite compounds; among them, 13 compounds have the same 3D structural motif as the perovskite metal nitrides, 7 have a 2D motif and one structure has a 1D motif. The vast majority of these structures contain single nitrogen atoms; exceptions are \( Pmma \)-Li2Ga3N6, \( P6/mmm \)-Li2Be2N6 and \( P\bar{1} \)-LiMgN4, which contain groups (a), (i) and (o), according to Figure 32, respectively. In principle, it is quite possible that higher metal nitrides containing three or even more chemically different metals in their structural units also exist, but no information on this subject has been reported so far.
4. Prospects of application of higher metal nitrides
While considering the possibility of application of higher metal nitrides, one should first of all imply their use as the key components of HEDMs; this possibility is considered below in Section 4.1. Along with this, other fields of practical application for HMNs are also emerging; they are discussed in more detail in Section 4.2.
4.1. Higher metal nitrides as HEDMs
The most significant application for HMNs of various s-, p-, d- and f-elements considered in the review is the use as components of high energy density systems and materials. The point is that these metal-containing compounds decompose very easily to give dinitrogen gas N2, and this reaction is accompanied by a pronounced increase in the volume (and, hence, in the entropy) of the reaction system and a significant heat evolution. This aspect of their practical use has been discussed in many of the studies that have already been cited in Section 2. Here, an important circumstance deserves mention: all these studies were concerned only with homometallic higher nitrides; in any case, none of the heterometallic nitrides mentioned in Section 3 has been so far considered for this purpose.
The key parameters that should be taken into account to evaluate high-energy chemical compounds usually include the mass (Eg) and volumetric (Ev) energy density and the detonation velocity (D)[215]. Particularly these values were determined in the studies related to the search for new HEDMs based on higher metal nitrides. However, most often, Eg, Ev and D were not determined experimentally, but were found from the results of crystal structure calculations, most often, by the CALYPSO method[216], which were treated according to a reported method[95] using empirical equations described by Kamlet and Jacobs[217]. Data on the detonation characteristics of some higher nitrides of various s-, p-, d- and f-elements are summarized in Table 9. Although the amount of data still obviously needs to be increased, it can be stated that quite a few compounds considered in this review are not inferior to traditional HEDM, 2,4,6-trinitrotoluene (TNT), in their detonation performance and often are even superior to TNT in one, two or even all three characteristics indicated in Table 9. Some of these compounds also surpass, in some detonation performance characteristics, more powerful explosives based on organic compounds such as hexogen [RDX, (CH2)3N3(NO2)3] and octogen [HMX, (CH2)4N4(NO2)4]. Among these HMNs, YN10 with \( Imm2 \) FSGS especially stands out in the Eg value (9.33 kJ g–1)[99], which is only slightly lower than that of so-called cubic gauche nitrogen \( cg \)-N (9.70 kJ g–1)[53]. In this regard, it should be noted that other higher yttrium nitrides reported in the literature, namely, YN4, YN5, YN6, YN7, YN8 and even YN15, have much lower Eg values than YN10. Nevertheless, the authors of almost every paper devoted to these compounds note the possibility of their use as HEDMs. The next compound in the order of decreasing Eg is \( P\bar{1} \)-ZnN10 (Eg = 6.57 kJ g–1)[162]; Eg > 6.0 kJ g–1 values were found also for \( P2_{1}/c \)-BeN4 (6.35 kJ g–1)[53], \( I4_{2}d \)-AlN5 (6.14 kJ g–1)[177], \( P\bar{3}1c \)-CoN8 (6.14 kJ g–1)[146] and \( P\bar{1} \)-CsN5 (6.03 kJ g–1)[42, 52]. The situation drastically changes if metal nitrides included in Table 9 are arranged in the order of decreasing two other parameters: Ev and D. The highest Ev value is inherent in \( P\bar{1} \)-FeN5 (19.89 kJ cm–3), while the highest D is characteristic of \( C2/m \)-CdN6 (17.79 km s–1).
However, the Eg values of these compounds are rather modest (4.32 and 3.82 kJ g–1) compared to other HMNs (see Table 9); hence, no clear correlations can be followed between the key detonation characteristics (Eg, Ev and D). It is also noteworthy that there is also no correlation between these characteristics and the mass fraction of nitrogen, although this could be expected at least for metal nitrides that are formed by atoms of one and the same metal. However, the greater part of these HEDMs are products of the mind of physicists and theoretical chemists; hence the data presented in Table 9 are still waiting to be confirmed in real experiments. Nevertheless, it is necessary to mention a study by Zhang et al.[112], who theoretically demonstrated the possibility of existence of higher nitrides ZrN10 and HfN10 formed at moderate pressures (in the case of HfN10, it is 23 GPa).
From the chemical standpoint, decomposition of HMNs looks rather simply as regards the range of final reaction products, which include dinitrogen gas and a solid phase consisting of either the corresponding elemental metal, or another nitride of this metal with a much lower nitrogen content, or a mixture of these components. In some studies, this process was examined in considerable detail. Zhang et al.[63] analyzed the destruction of MN10 (M = Mg, Ca, Sr, Ba). According to the results of this study, these HMNs, which are nothing other than metal bis(pentazolates), decompose according to the pathway MN10 → MN8 → MN6 involving stepwise elimination of one N2 molecule (Figure 39). The activation energies for each step of this reaction (Ea,1, Ea,1’, Ea,2 and Ea,2') were determined. In particular, in the case of MgN10, these values were 97.7, 238.3, 99.9 and 241.0 kJ mol–1 and those for BaN10 were 106.9, 202.5, 108.8 and 201.7 kJ mol–1, respectively. The Ea,1 and Ea,2 values tend to regularly increase in the series Mg – Ca – Sr – Ba, whereas the Ea,1' and Ea,2' values, conversely, decrease[63]. Note that the \( \Delta_{r} H° \) values for the reactions MN10 → M + 5N2 and MN8 → M + 4N2 monotonically increase in this series of metals, while \( \Delta_{r} G° \) pass through a maximum at SrN10 (Table 10). As was to be expected, these values are all negative; since the entropies \( \Delta_{r} S° \) for both of the above reactions are a priori positive, each reaction is virtually irreversible for any of the metals. One more publication considering the destruction of triazides AlN9 and GaN9 appeared[83] almost at the same time as the publication cited above[63]. According to Cheng et al.[83], out of the two possible planar M(N3)3 structures (\( C_{3h} \) and \( C_{S} \)), where M = Al, Ga, the former is energetically the most favourable for both of these molecules, the ground state of which is spin singlet.
A study of the potential energy surface of the unimolecular decomposition of planar azides AlN9 and GaN9 both with \( C_{3h} \) and \( C_{S} \) symmetry showed that AlN9 decomposes by a two-step mechanism, with the reaction Al(N3)3 → Al(N3)2N + N2 being the first step and Al(N3)2N → AlN3 + 2N2 being the second step. Dissociation of GaN9 proceeds in one step: Ga(N3)3 → GaN3 + 3N2. It is characteristic that AlN3 has a linear structure (Figure 40), whereas GaN3 is angular (Figure 41)[83]. In the authors’ opinion, these data may be useful for the understanding of the mechanisms of decomposition of Group 13 element triazides and the possible mechanism of formation of MN films. It is also necessary to mention a paper by Choi et al.[107], who considered the destruction of tris(pentazolates) M(N5)3 (M = Sc, Y) and tetrakis(pentazolates) M(N5)4 (M = Ti, Zr, Hf) and showed that for the above-mentioned higher nitrides M(N5)3, this reaction consists of three steps:
Each of the steps is accompanied by intramolecular degradation of one pentazolate anion to the azide anion N3– and dinitrogen. Decomposition of the above-mentioned HMNs with the composition M(N5)4 proceeds in a similar way, but includes four steps to give finally the corresponding tetraazides M(N3)4. As was to be expected, all these reactions are characterized by \( \Delta_{r} H° < 0 \) and \( \Delta_{r} S° > 0 \) and, hence, they are virtually irreversible. Note in this respect that these conclusions were drawn[63, 83, 107] on the basis of quantum chemical calculations using various DFT methods and more advanced CCSD (T) method. No other studies that consider in detail the specific features of HMN decomposition have yet been published, although some mentions of this issue can be found in some publications[67, 174, 218].
4.2. Other applications of higher metal nitrides
Another possible application of HMNs is their use as matrices for the preparation of polynitrogens containing large numbers of nitrogen atoms, which, in turn, can also be utilized as highly efficient HEDMs. This application can be considered as a sort of extension for the application that has been already discussed in Section 4.1. This aspect was also addressed in a number of studies[219-222] in which the CALYPSO method was used to predict the formation of polynitrogen from dinitrogen N2 molecules for various azides of four Group 1 s-elements, namely, LiN3[219], NaN3[220], KN3 (see [221]) and CsN3 (see [222]) upon generation of high pressure in the reaction system. Eremets et al.[220] noted the formation of nitrogen atom clusters and polymer networks in the pressure range of 19 – 120 GPa accompanied by darkening and turbidity of the initial matrix sample. It was also noted that this process is enhanced by laser heating and application of shear strain to the reaction system. The polymer forms can be retained upon decompression in a diamond anvil cell; however, under ambient conditions, in particular, at standard ambient temperature and pressure (i.e., T = 298.16 K and P = 101325 Pa), they are converted rather rapidly into the initial azide and other new phases. Wang et al.[222] used the CsN3 matrix at pressures of 6, 13 and 51 GPa and thus identified four stable phases of nitrogen atoms with \( I4/mcm \), \( C2/m \), \( P2_{1}/m \) and \( P\bar{1} \) space groups, respectively. The first three phases contain linear chains consisting of three nitrogen atoms, whereas the last one, which is the most stable among them, contains a set of longer (zigzag-like) chains, each consisting of eight nitrogen atoms. Wei et al.[53] used the higher nitride BeN4 as a matrix for nitrogen polymerization. Note a study by Zhang et al.[112], who indicated the applicability of higher nitrides ZrN10 and HfN10 as a matrix for stabilizing polynuclear compounds of nitrogen.
One more important area related to the practical application of the compounds discussed in the review is their potential use as superhard materials (SHMs). However, there is much less information on this subject in the literature than the structural and thermodynamic data for these compounds. Nevertheless, the applicability of HMNs of various s-, p-, d- and f-elements as SHMs has been considered in the literature[223-230], in particular, in some of the publications discussed above (for example,[28, 54, 77, 79, 126, 134, 150]). Li et al.[54] noted the ultrahigh mechanical strength of Be2N6 (about 200 J m–2). The authors attributed this fact to the presence of twelve classical two-centre two-electron (2c–2e) σ-bonds and five multicentre (6c – 2e) π-bonds, and ten π-electrons in the Be2N6 crystal unit cell, which corresponds the Hückel rule [4n + 2] at n = 2; therefore, the Be2N6 monolayer is aromatic. (In this regard, we would like to note that this explanation seems rather strange, because no correlation between the aromaticity and strength has been noted so far in either theoretical or experimental studies). High Vickers hardness (HV, GPa) values were, in particular, noted for HMNs such as CrN4 (57.2 GPa)[28], AlN5 (37.1 GPa)[77], WN4, ReN4 and OsN4 (64.3, 64.5 and 67.3 GPa, respectively)[126]. A study devoted to the binuclear heterometallic nitride ThTaN3 indicated[182] that metal nitrides (as well as oxide nitrides) may be of interest as proton-conducting ceramics. It cannot be ruled out either that some of these compounds could even act as high-temperature superconductors, although no detailed information regarding this possibility has been presented in the literature so far.
5. Conclusion
Detailed analysis of the data available from the literature on higher metal nitrides (HMNs) provides the following considerations some of which may seem quite unusual. To begin with, the major array of publications devoted to HMNs (as indicated in the Introduction, we assign to this class only those metal compounds with nitrogen the structural units of which contain at least three nitrogen atoms per metal atom) has appeared in the last 15 – 20 years (i.e., starting approximately with 2005). These publications reflect the results of both experiments and quantum chemical calculations; furthermore, the experimental works in this area refer, most often, to the high- pressure physicochemistry. As regards purely theoretical studies, it is noteworthy that many of them are very loaded (if not overloaded) with a wide variety of scientific data, including not only structural and/or thermodynamic data related to higher metal nitrides (e.g., Raman and phonon dispersion spectra, phonon density of states, localization of electron states, etc., which, however, are of interest for solid-state physicists rather than for chemists and, hence, are not mentioned in this review). In addition, there is a paradoxical situation that the number of studies in which the above-mentioned compounds were considered only theoretically has markedly increased with time up to the present day, whereas the number of works in which these compounds were synthesized in one way or another in an experiment has, conversely, decreased. As a consequence, currently, the array of theoretical studies devoted to HMNs appears to be much larger than the array of studies devoted to the synthesis of these compounds and identification of their macroscopic physicochemical characteristics. The foregoing refers, however, to so-called homometallic HMNs containing nitrogen atoms and atoms of a single metal. Meanwhile, for heterometallic HMNs considered in Section 3, the situation is rather the opposite, as most of these compounds have been obtained experimentally, with these experimental works being mainly published before 2015. Among other reasons, this can be due to the clear trend, followed today by quite a number of chemists, towards giving preference, whenever possible, to predicting chemical reactions by various quantum chemical methods rather than to observing the reactions in a real experiment. Presumably, one of the most important reasons for this situation is the fact that at the currently available level of quantum chemical methods, these predictions may be highly reliable even without resorting to the experimental preparation of the desired compounds (especially in view of the fact that synthesis of compounds that are discussed in this review occurs under very specific conditions and requires both special equipment and highly skilled experimenters).
In this review, we barely discussed issues related to HMN synthesis, which is caused by at least two circumstances. First, as mentioned above, most of the studies addressing these exotic chemical compounds consider them only theoretically using modern computational methods to calculate the molecular and/or crystal structures; no experimental data or synthetic methods were mentioned in these publications. Second, in the relatively few studies in which the compounds were obtained experimentally, their synthesis was based, almost in all cases, on applying high pressure to binary reaction systems such as (metal + nitrogen), (metal azide + nitrogen), (metal pentazolate + nitrogen), with the physicochemical features of the synthesis being usually beyond the attention of researchers. Anyway, all publications on the problems indicated in the title of this review are original research papers. No studies in which the experimental and/or theoretical data on the whole range of metal elements (i.e., taking into account their subdivision into s-, p-, d- and f-elements accepted in modern general chemistry) would be integrated and systematized are currently available in the literature. Actually, the cause for this is unclear. Indeed, the available body of published data devoted to these exotic compounds seems to be quite sufficient to summarize them. This review is an attempt to perform this task. Nevertheless, at the present moment, we still have to admit that the scope and the total body of reliable experimental data related to the molecular and/or crystal structure, as well as to the synthesis of these compounds, are still quite small, and, therefore, a critical analysis that would show the efficacy, benefits and drawbacks of theoretical modelling seems to be premature. Nevertheless, it should be noted that in those relatively rare cases where the experimental data were compared with the results of DFT, CALYPSO and other calculations, the agreement between them was fairly good. In view of this important circumstance, there is reason to believe that the body of theoretical material that has already been gained will be confirmed in the future, and the compounds predicted theoretically will be prepared experimentally as well.
The following aspects related to the further development of the subject matter considered in this review can be highlighted:
1. Further development of methods for the synthesis of higher metal nitrides of s-, p-, d- and f-elements and methods for HMN stabilization under ambient conditions for relatively long periods of time.
2. Further improvement of theoretical computational methods for the above chemical compounds, first of all, the methods directed towards determination of the kinetics and mechanisms of reactions to prepare these compounds that could proceed at relatively low pressures and temperatures.
3. For the most part, the title compounds belong to the class of so-called high energy density materials (HEDMs), and many papers devoted to them focus on exactly this circumstance. This application, however, is not the only one, and ideas about other possible applications are already stated in some papers, for example, as stabilizers for polynitrogens and superhard compounds, and there are reasons to believe that this list could be markedly expanded. Therefore, it seems appropriate to search for new possible ways of using these products in human activity.
Thus, the improvement of synthetic routes to prepare these unique compounds and identification of the scope of their practical use seem to be a relevant challenge of the modern condensed-state and high-pressure physicochemistry as well as the chemical engineering of explosives; therefore, there is no doubt that they deserve close attention and further theoretical and experimental studies.
6. List of abbreviations and symbols
DFT — density functional theory;
FSGS — Fedorov space group of symmetry;
HEDM — high energy density material;
HMN — higher metal nitride;
SHM — superhard material.
References
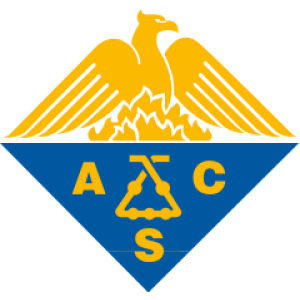
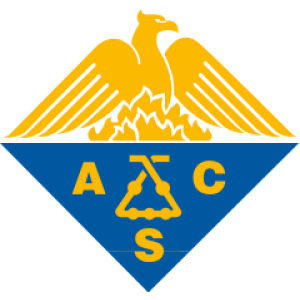
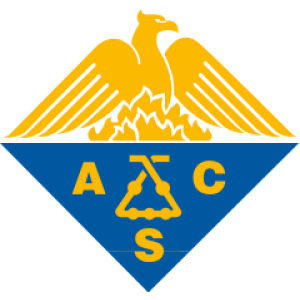
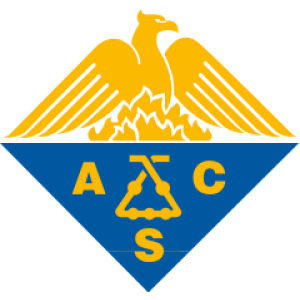
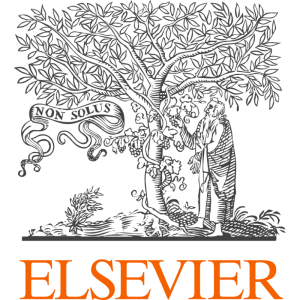
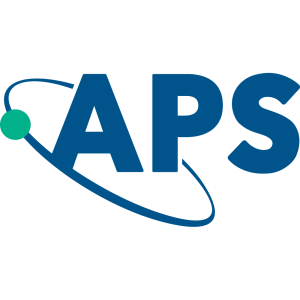
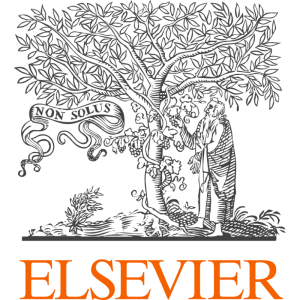
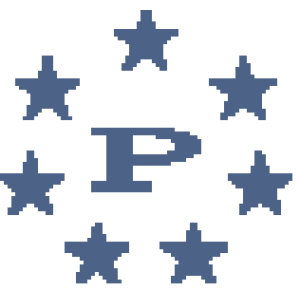
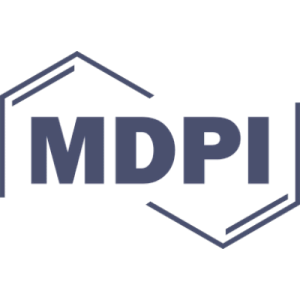
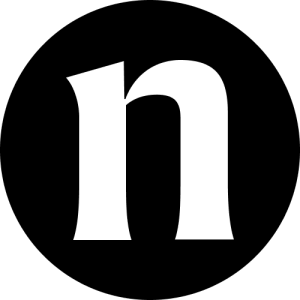
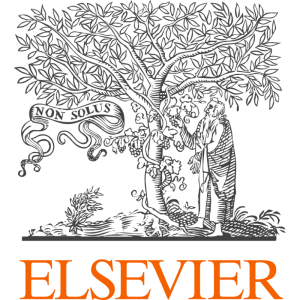
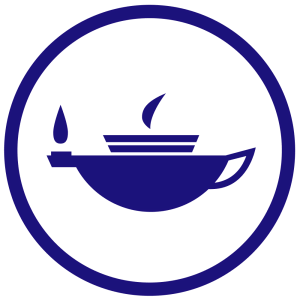
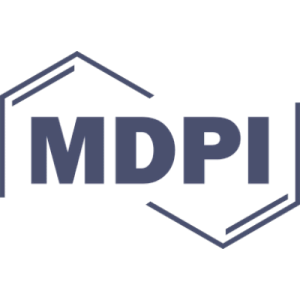
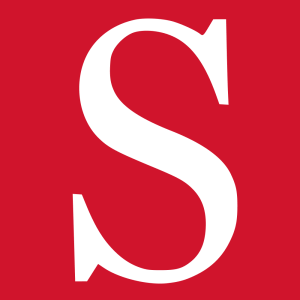
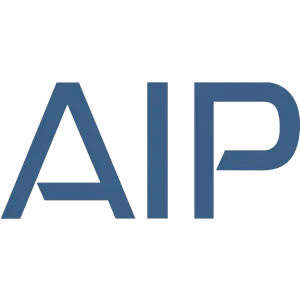
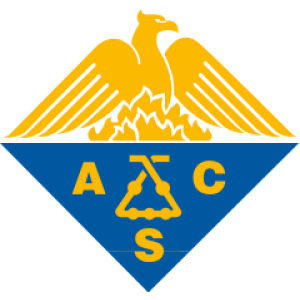
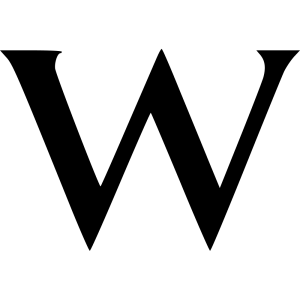
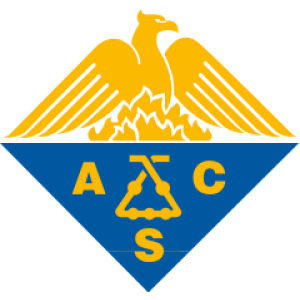
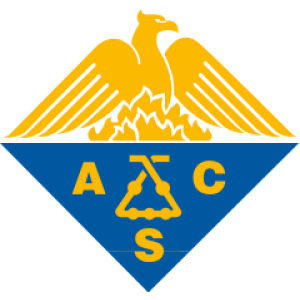
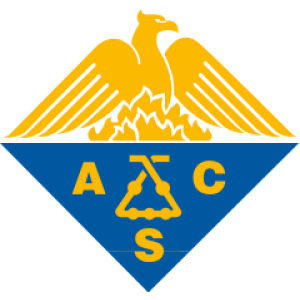
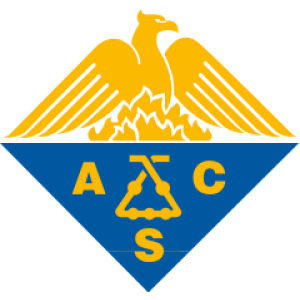
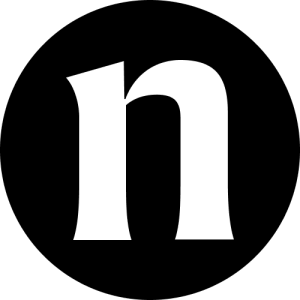
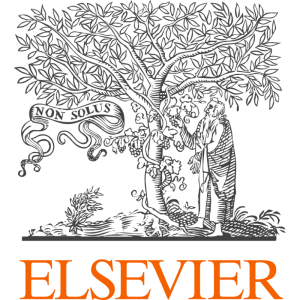
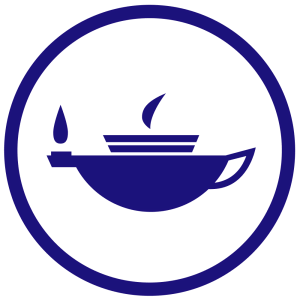
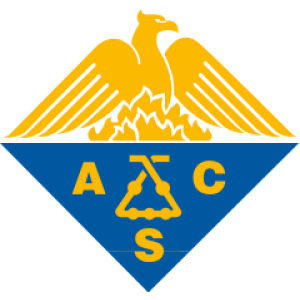
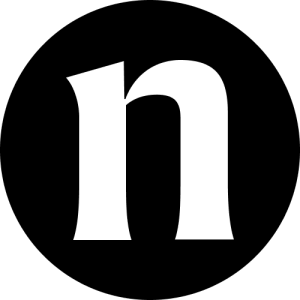
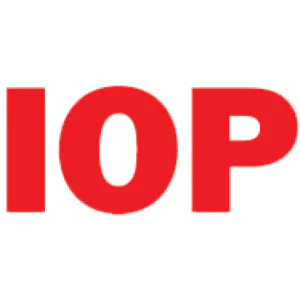
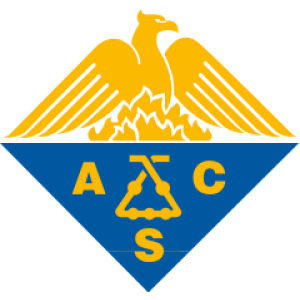
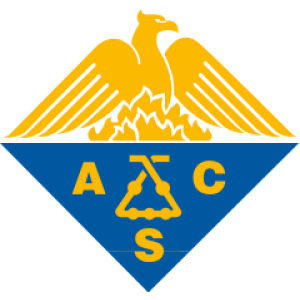
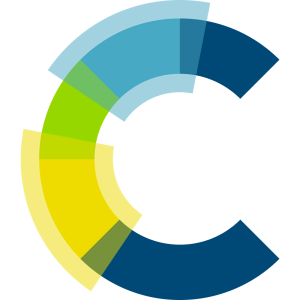
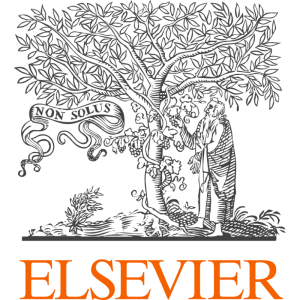
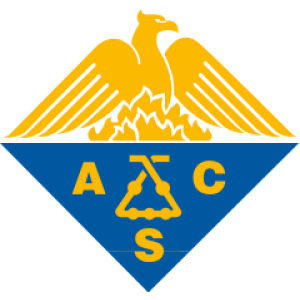
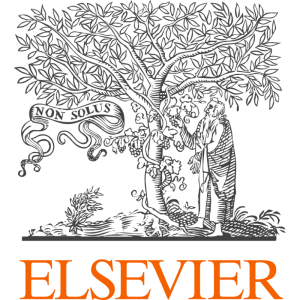
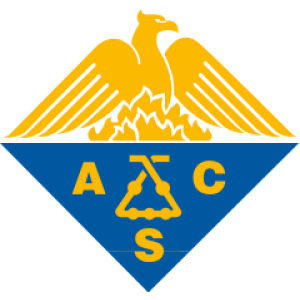
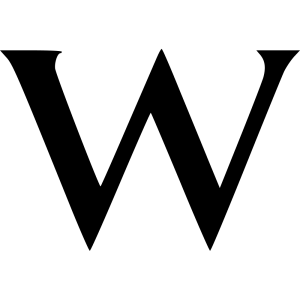
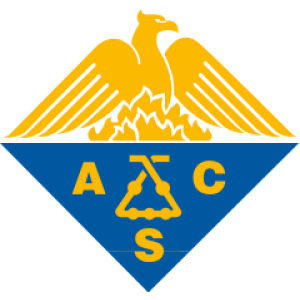
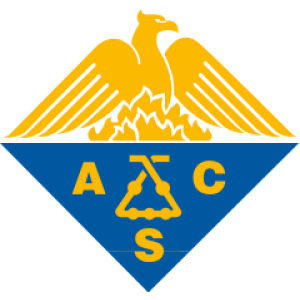
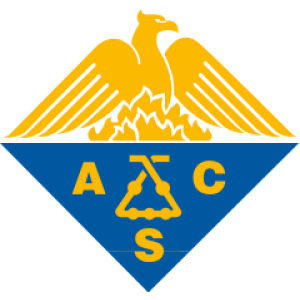
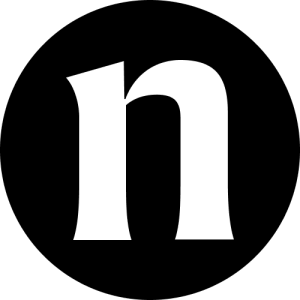
![Aromatic hexazine [N6]4− anion featured in the complex structure of the high-pressure potassium nitrogen compound K9N56](/storage/images/resized/voXLqlsvTwv5p3iMQ8Dhs95nqB4AXOG7Taj7G4ra_small_thumb.webp)
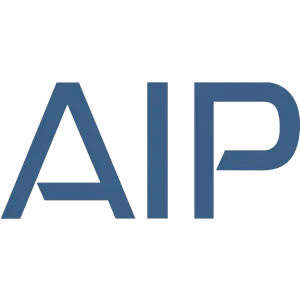
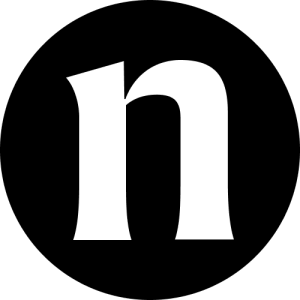
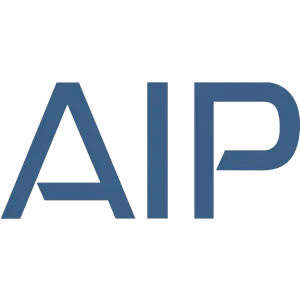
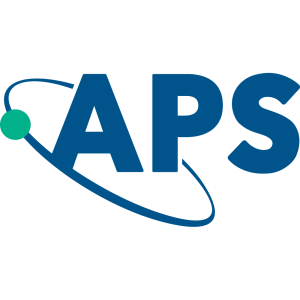
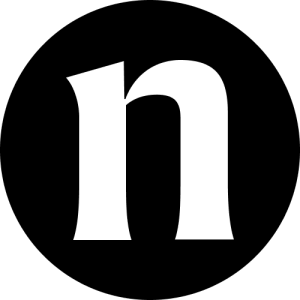
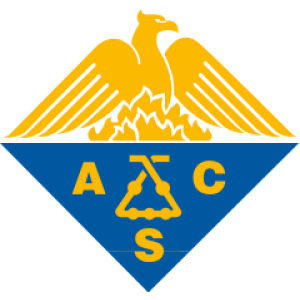
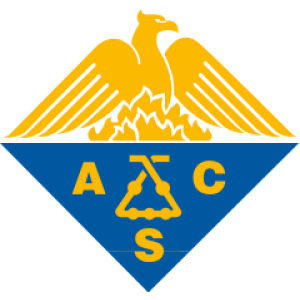
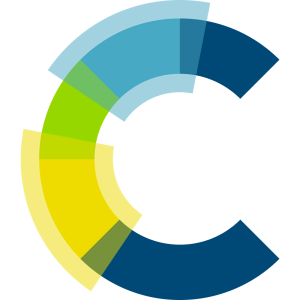
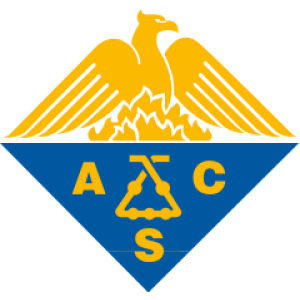
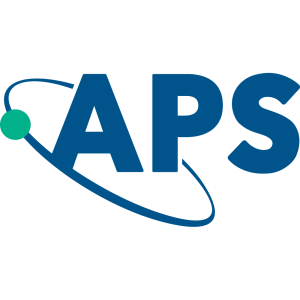
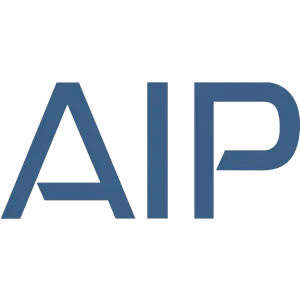
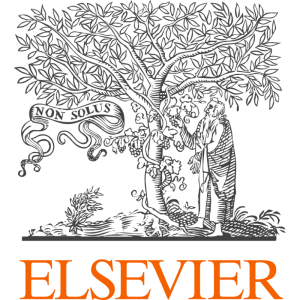
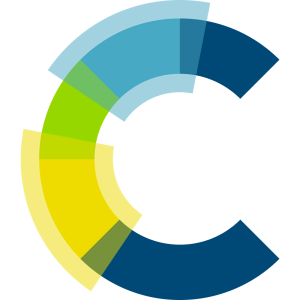
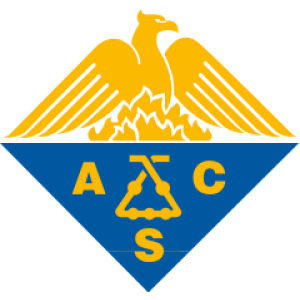
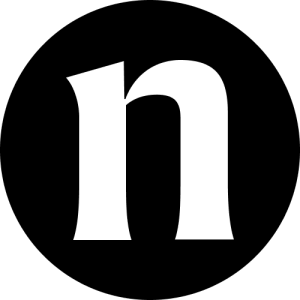
![Fully Active Nitrogen Energetic Chains Mg 2 (N 5 ) 2 N 2 [Mg 2 (N 5 ) 2 N 2 ] n under Ambient Conditions](/storage/images/resized/bRyGpdm98BkAUYiK1YFNpl5Z7hPu6Gd87gbIeuG3_small_thumb.webp)
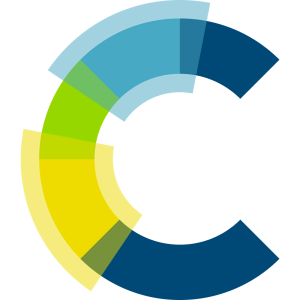
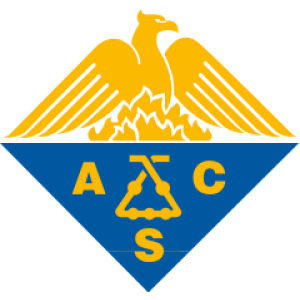
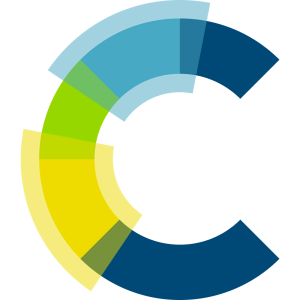
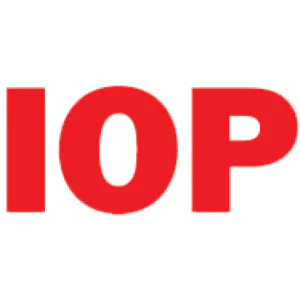
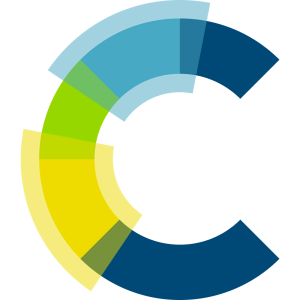
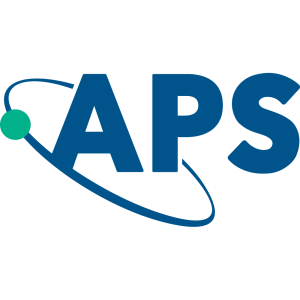
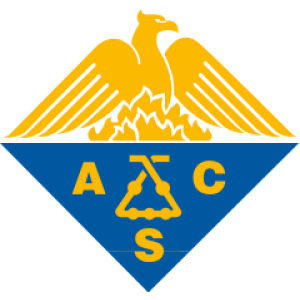
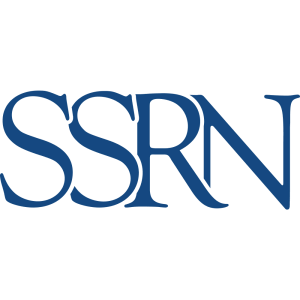
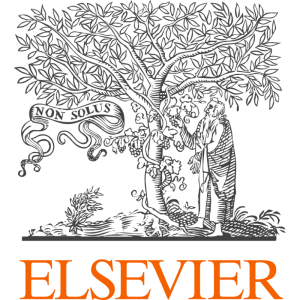
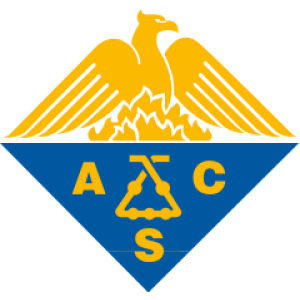
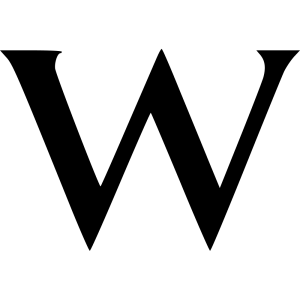
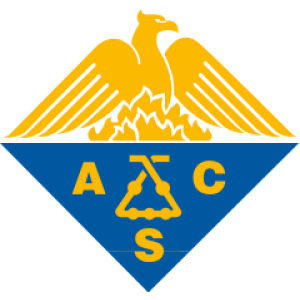
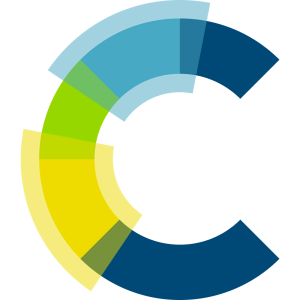
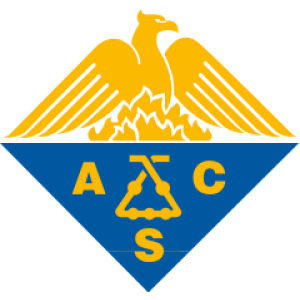
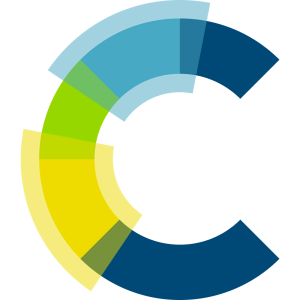
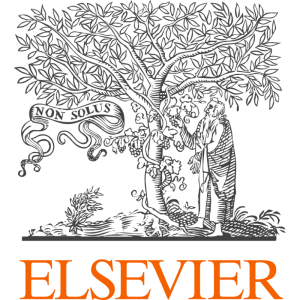
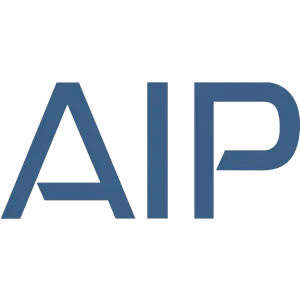
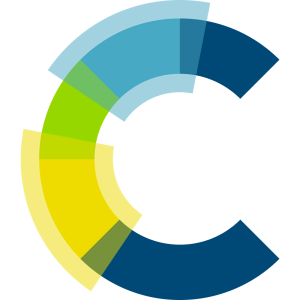
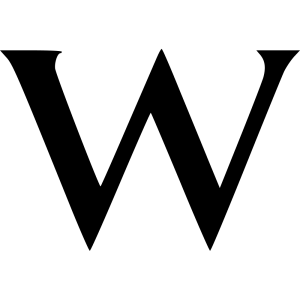
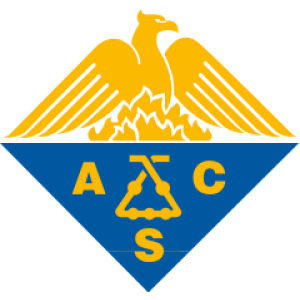
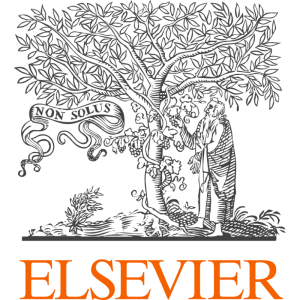
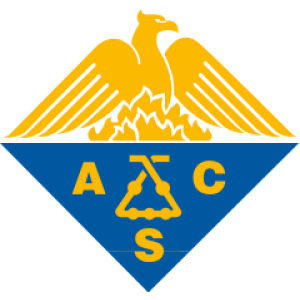
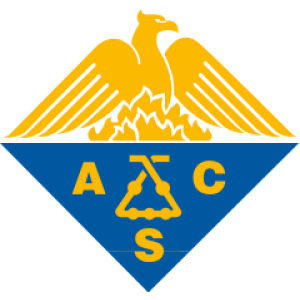
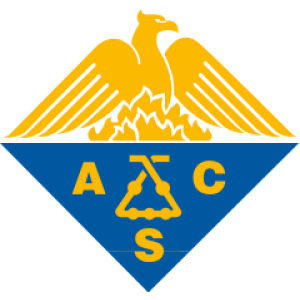
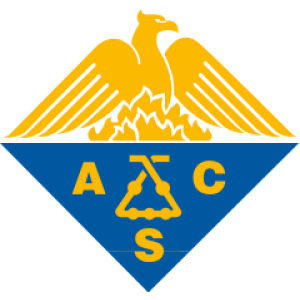
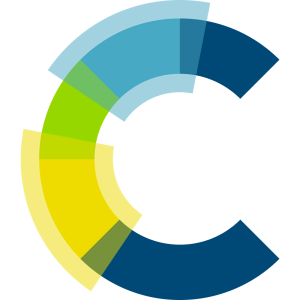
![Polyazide Chemistry: Preparation and Characterization of As(N3)5, Sb(N3)5, and [P(C6H5)4][Sb(N3)6]](/storage/images/resized/bRyGpdm98BkAUYiK1YFNpl5Z7hPu6Gd87gbIeuG3_small_thumb.webp)
![Binary Bismuth(III) Azides: Bi(N3)3 , [Bi(N3)4]−, and [Bi(N3)6]3−](/storage/images/resized/bRyGpdm98BkAUYiK1YFNpl5Z7hPu6Gd87gbIeuG3_small_thumb.webp)
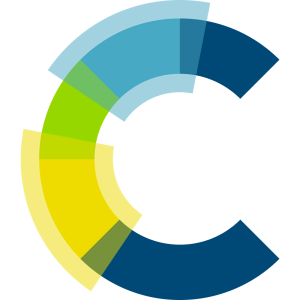
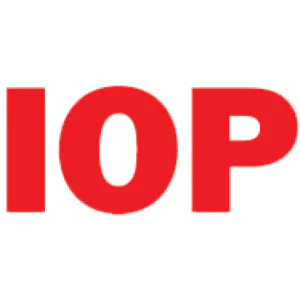
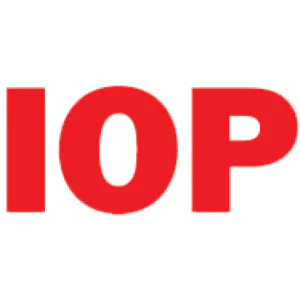
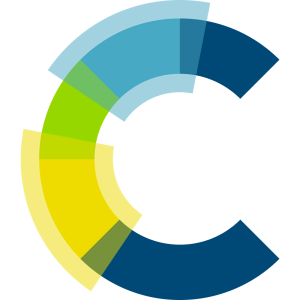
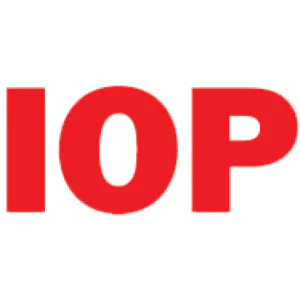
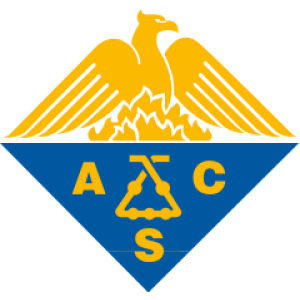
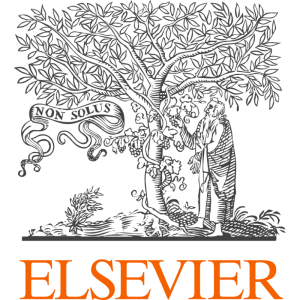
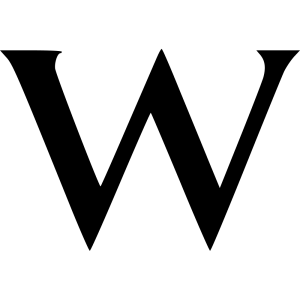
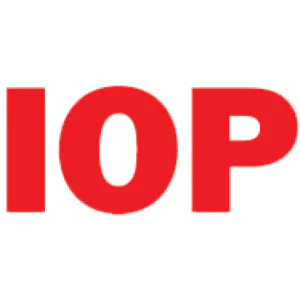
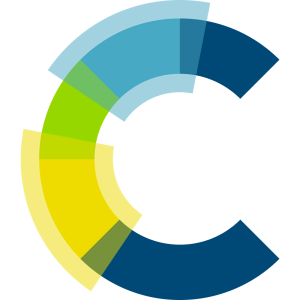
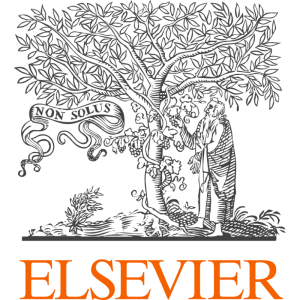
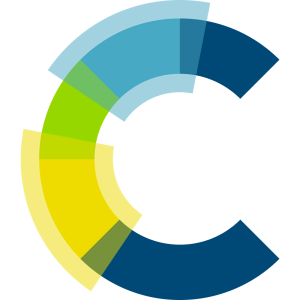
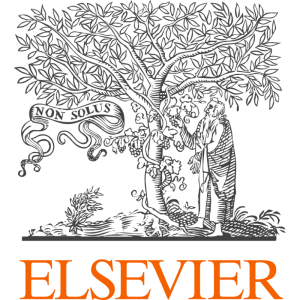
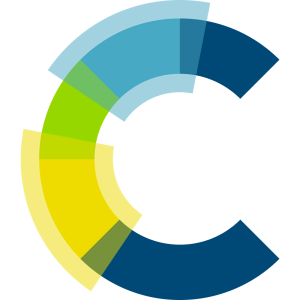
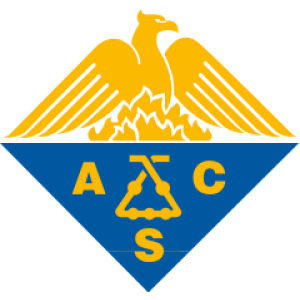
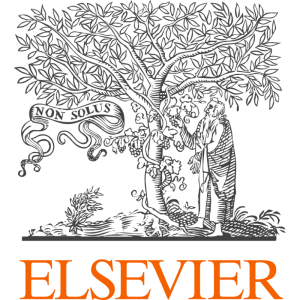
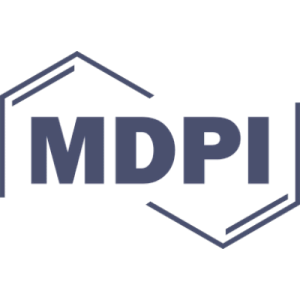
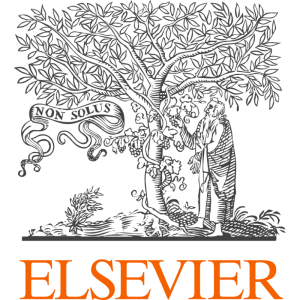
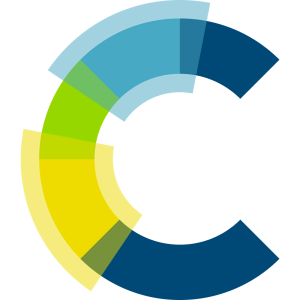
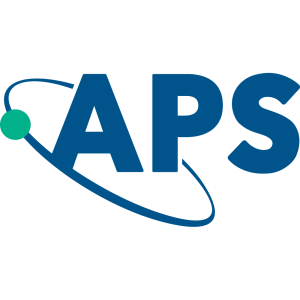
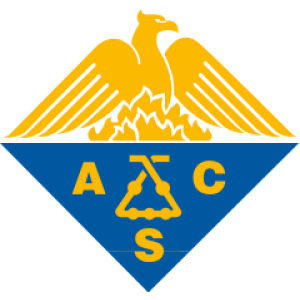
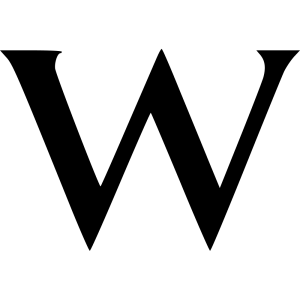
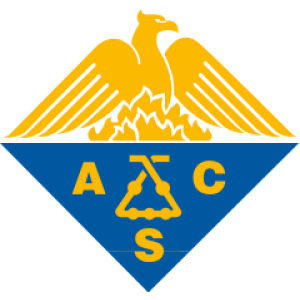
![The Syntheses and Structure of the Vanadium(IV) and Vanadium(V) Binary Azides V(N3)4, [V(N3)6]2−, and [V(N3)6]−](/storage/images/resized/bRyGpdm98BkAUYiK1YFNpl5Z7hPu6Gd87gbIeuG3_small_thumb.webp)
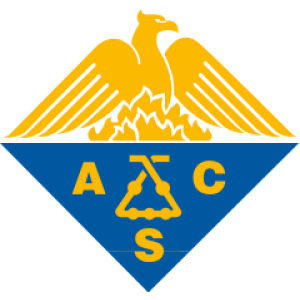
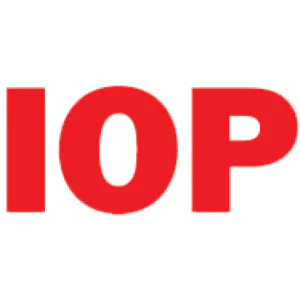
![Experimental Evidence for Linear Metal–Azido Coordination: The Binary Group 5 Azides [Nb(N3)5], [Ta(N3)5], [Nb(N3)6]−, and [Ta(N3)6]−, and 1:1 Acetonitrile Adducts [Nb(N3)5(CH3CN)] and [Ta(N3)5(CH3CN)]](/storage/images/resized/bRyGpdm98BkAUYiK1YFNpl5Z7hPu6Gd87gbIeuG3_small_thumb.webp)
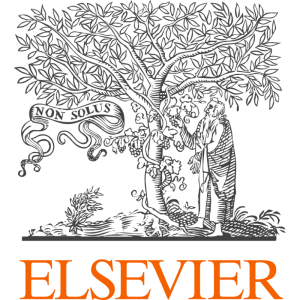
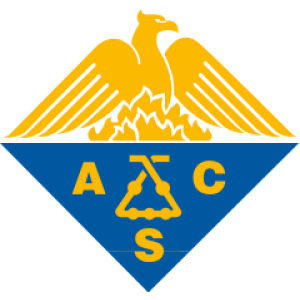
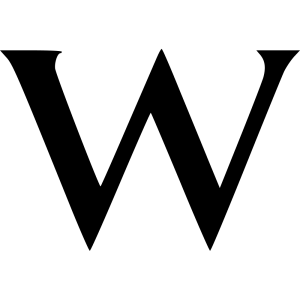
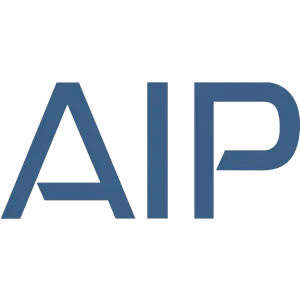
![Polyazide Chemistry: The First Binary Group 6 Azides, Mo(N3)6, W(N3)6, [Mo(N3)7]?, and [W(N3)7]?, and the [NW(N3)4]? and [NMo(N3)4]? Ions](/storage/images/resized/bRyGpdm98BkAUYiK1YFNpl5Z7hPu6Gd87gbIeuG3_small_thumb.webp)
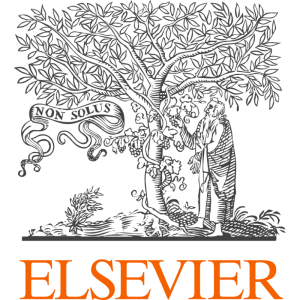
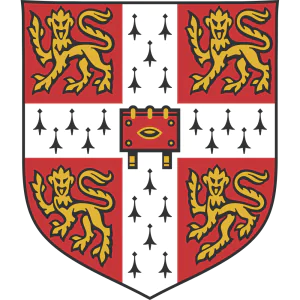
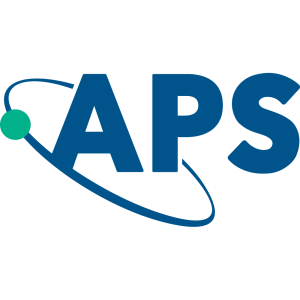
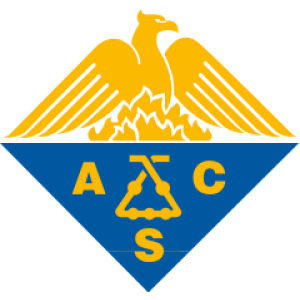
![Dual Functions of Water in Stabilizing Metal-Pentazolate Hydrates [M(N5)2(H2O)4]·4H2O (M = Mn, Fe, Co, and Zn) High-Energy-Density Materials](/storage/images/resized/iLiQsFqFaSEx6chlGQ5fbAwF6VYU3WWa08hkss0g_small_thumb.webp)
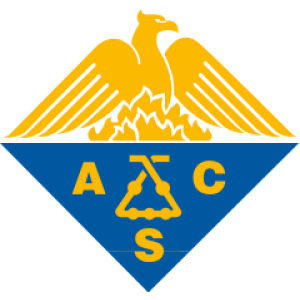
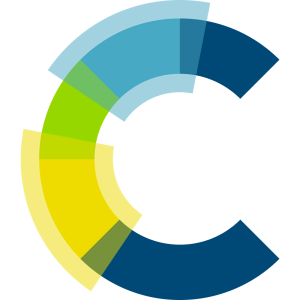
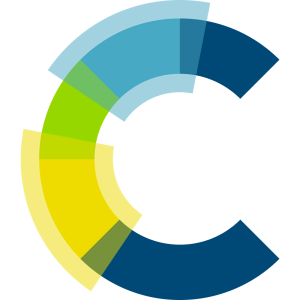
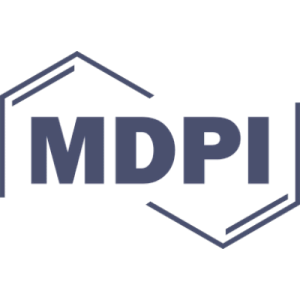
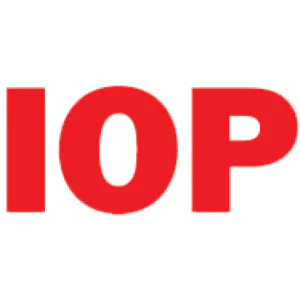
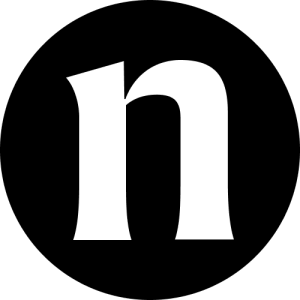
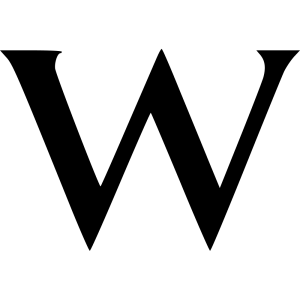
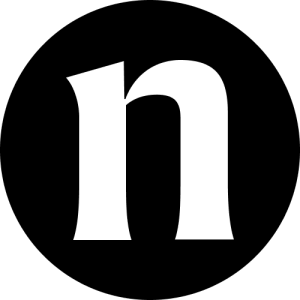
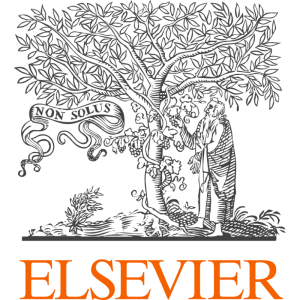
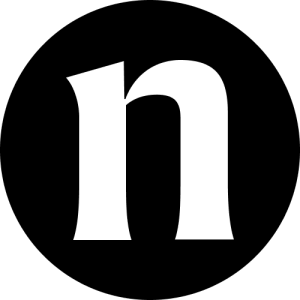
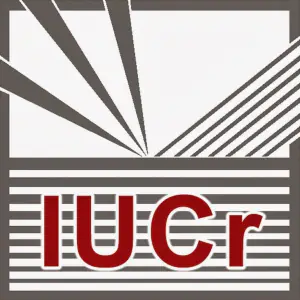
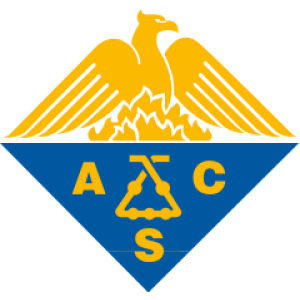
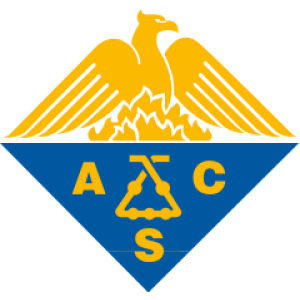
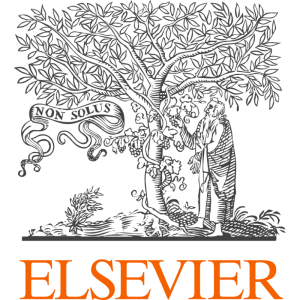
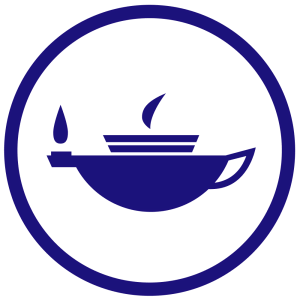
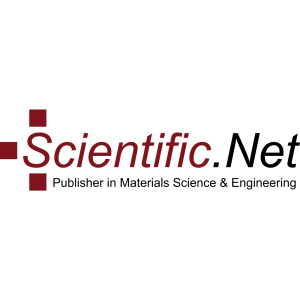
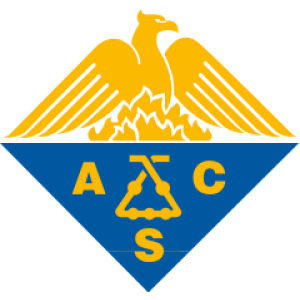
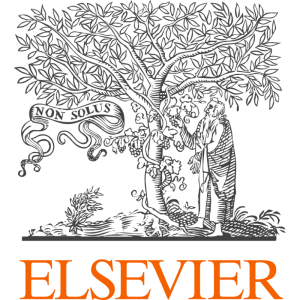
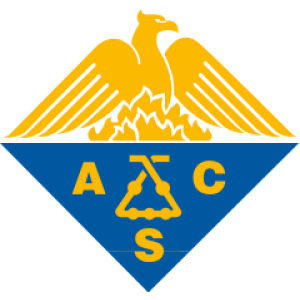
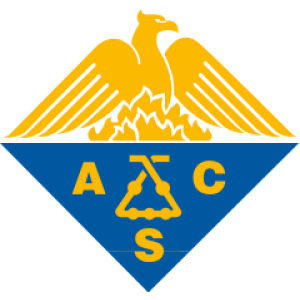
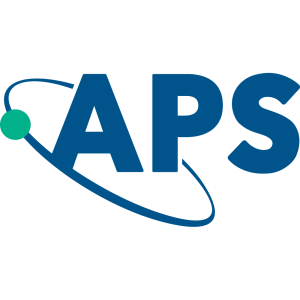
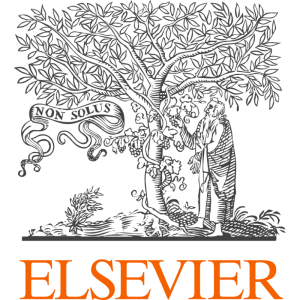
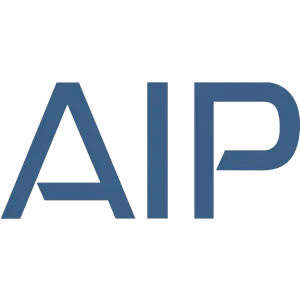
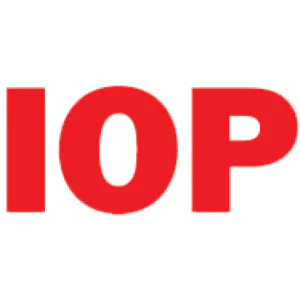
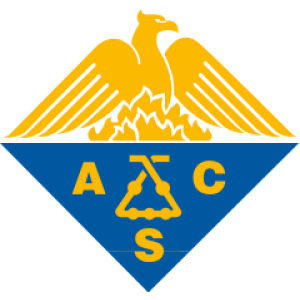
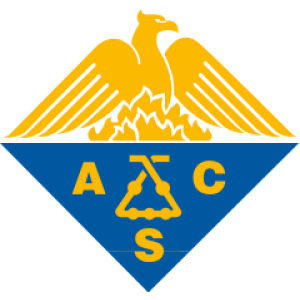
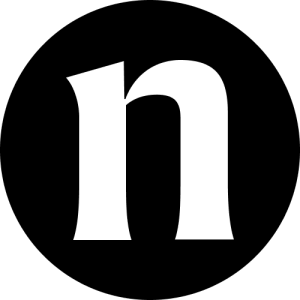
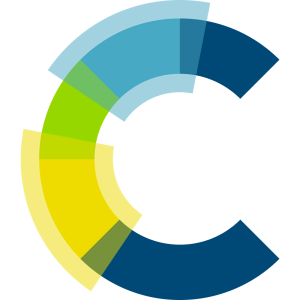
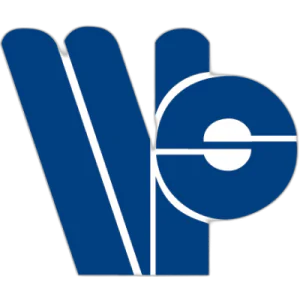
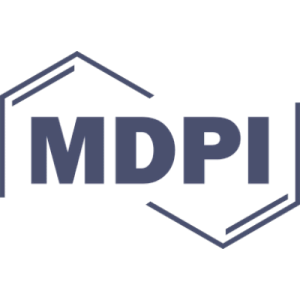
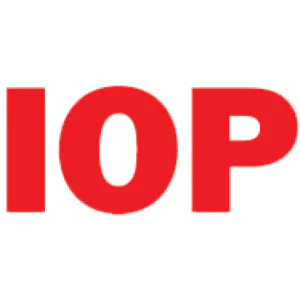
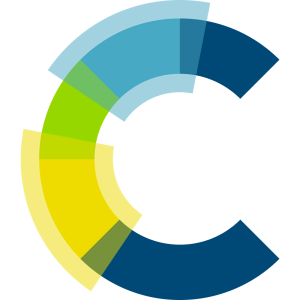
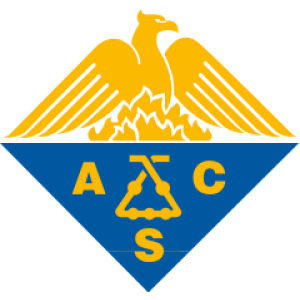
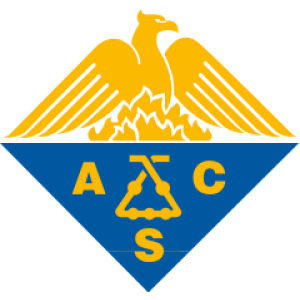
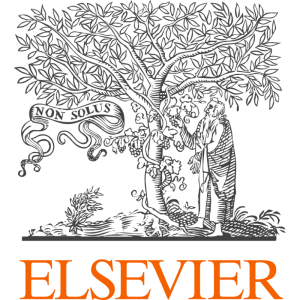
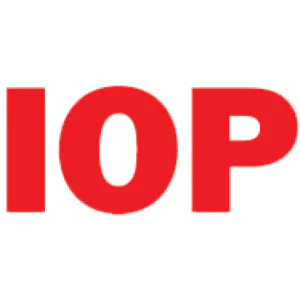
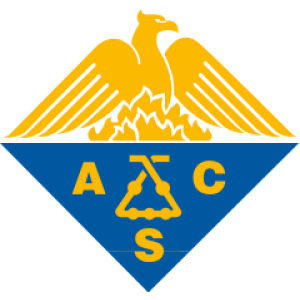
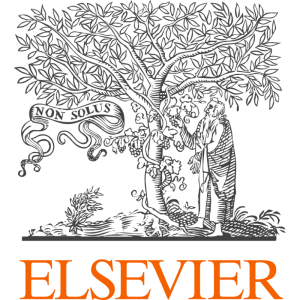
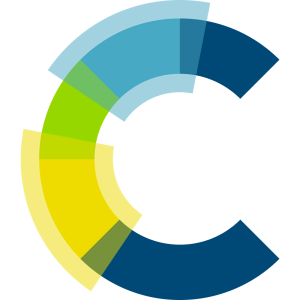
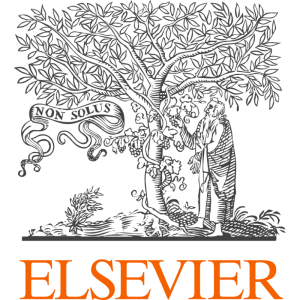
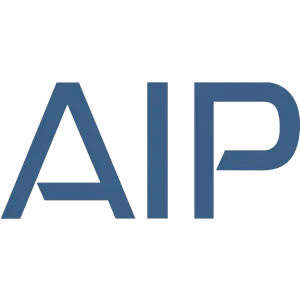
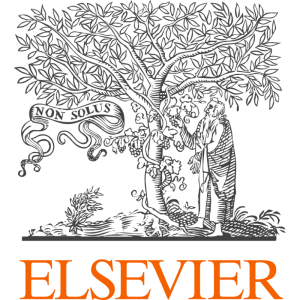
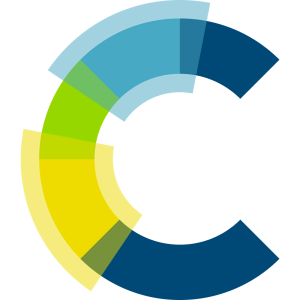
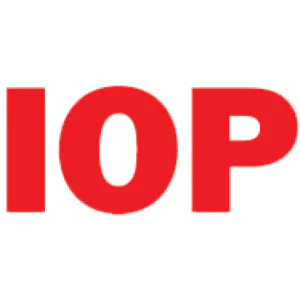
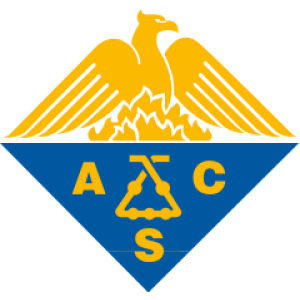
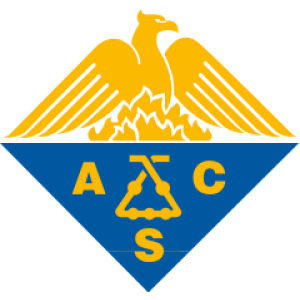
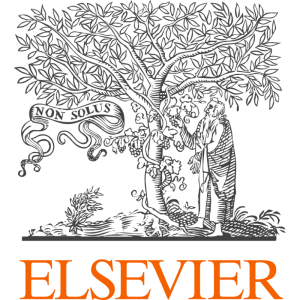
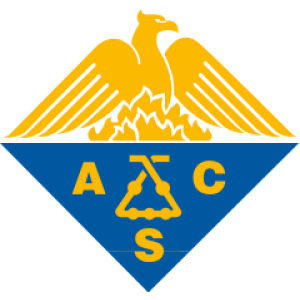
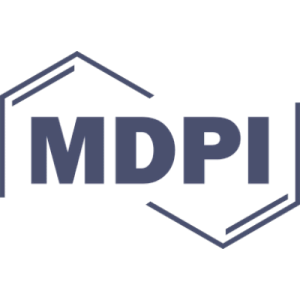
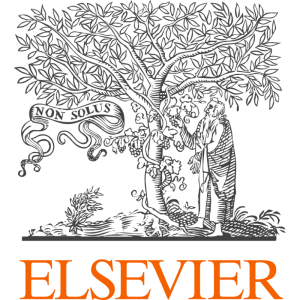
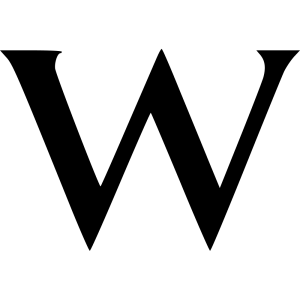
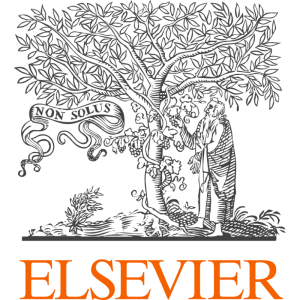
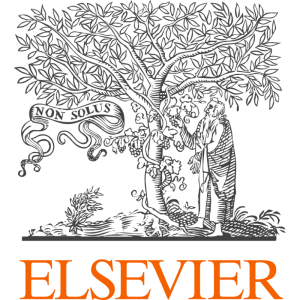
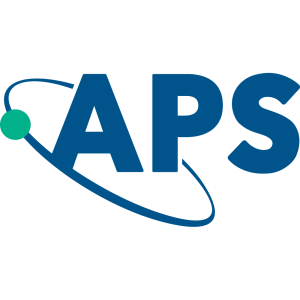
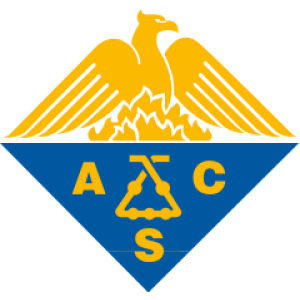
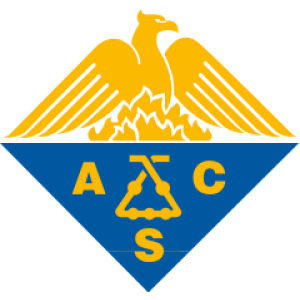
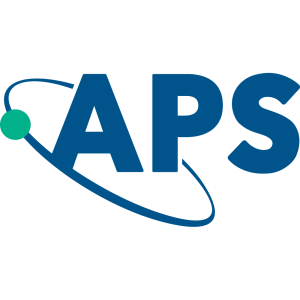
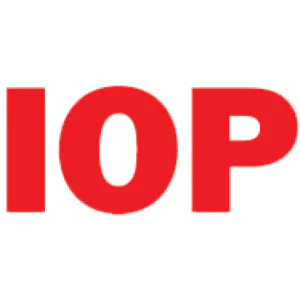
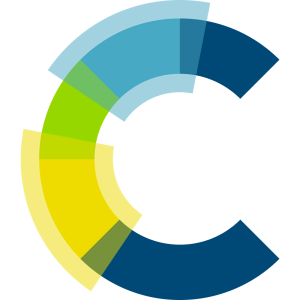
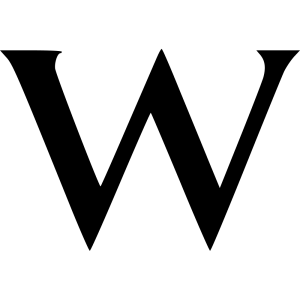
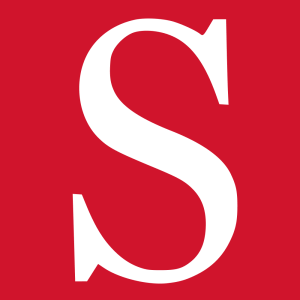
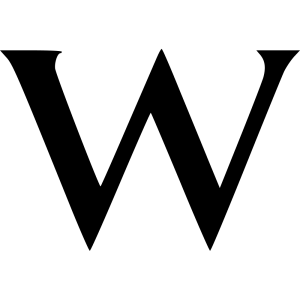
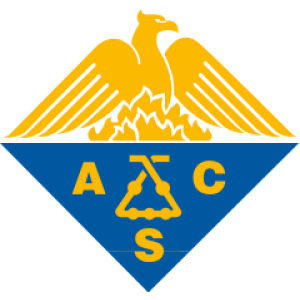
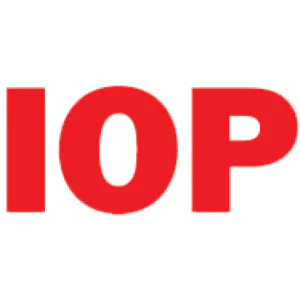
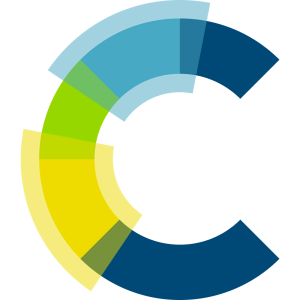
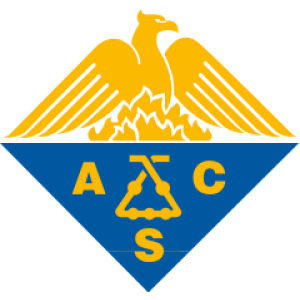
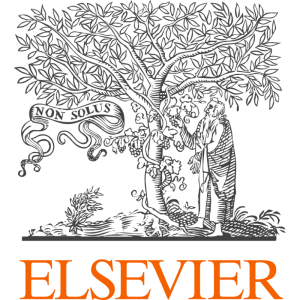
![Synthesis and Structure of Ba[ZrN2] and Ba2[NbN3]](/storage/images/resized/bRyGpdm98BkAUYiK1YFNpl5Z7hPu6Gd87gbIeuG3_small_thumb.webp)
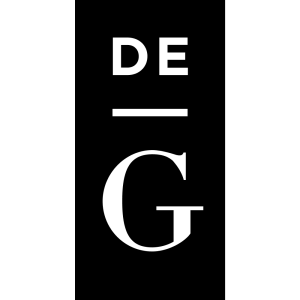
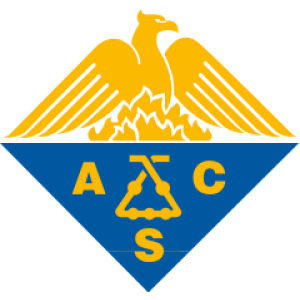
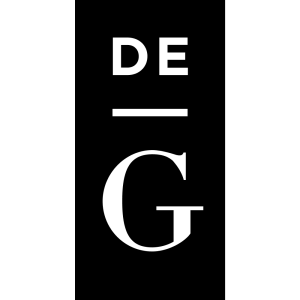
![XAS spectra of Ce2[MnN3] at the Ce-M4,5, Ce-L3, Mn-L2,3 and N-K thresholds](/storage/images/resized/GDnYOu1UpMMfMMRV6Aqle4H0YLLsraeD9IP9qScG_small_thumb.webp)
![Crystal structure of dicalcium trinitrido monovanadate(V), Ca2[VN3]](/storage/images/resized/3SpVxcYL33bOvPq4sHxJLH2NeKNeDloahSUpNiO4_small_thumb.webp)
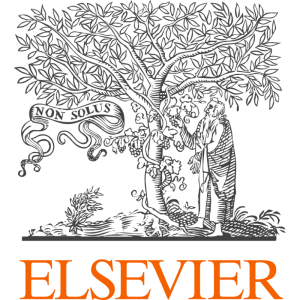
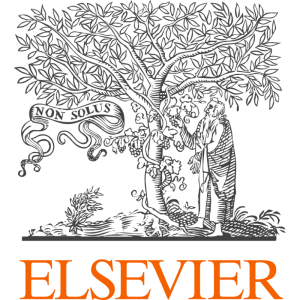
![Ce2[CrN3]: Single Phase Synthesis and Characterization of a Nitridochromate(I)](/storage/images/resized/bRyGpdm98BkAUYiK1YFNpl5Z7hPu6Gd87gbIeuG3_small_thumb.webp)
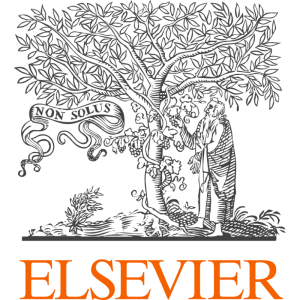
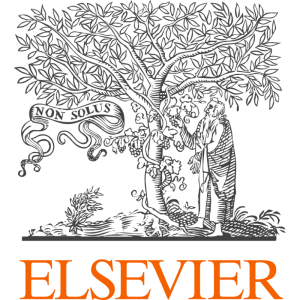
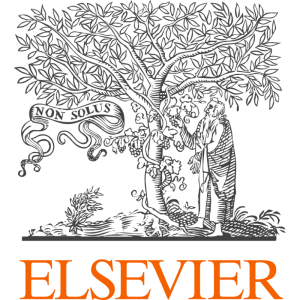
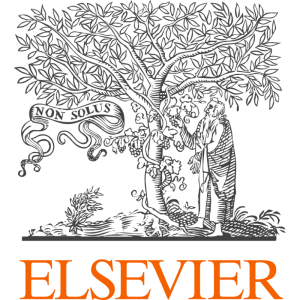
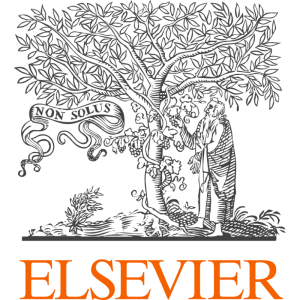
![High-Pressure Synthesis and Characterization of Li2Ca3[N2]3—An Uncommon Metallic Diazenide with [N2]2– Ions](/storage/images/resized/iLiQsFqFaSEx6chlGQ5fbAwF6VYU3WWa08hkss0g_small_thumb.webp)
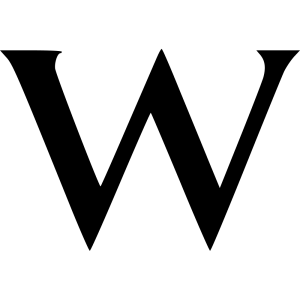
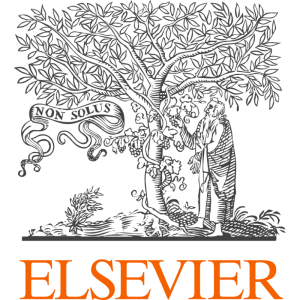
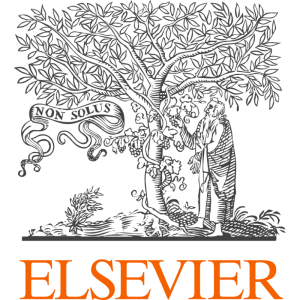
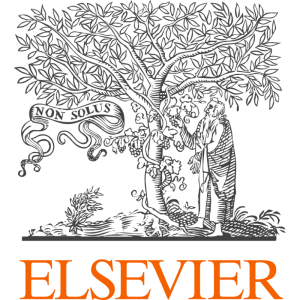
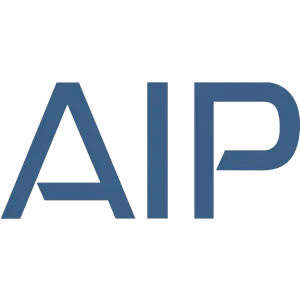
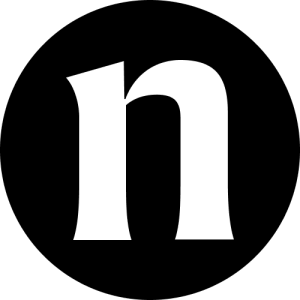
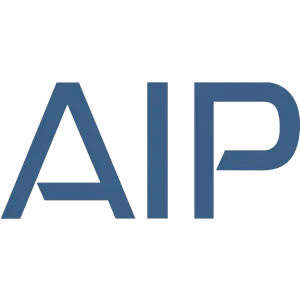
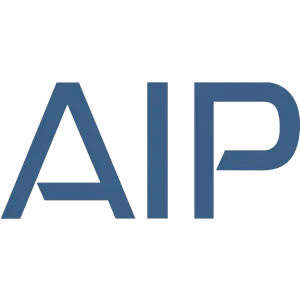
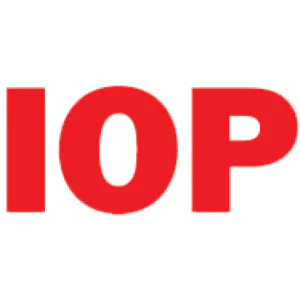
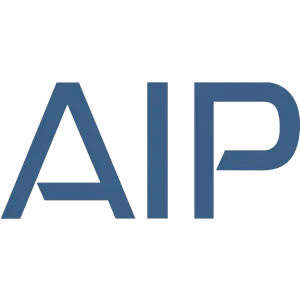
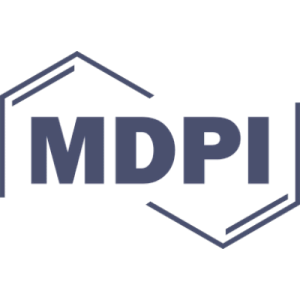
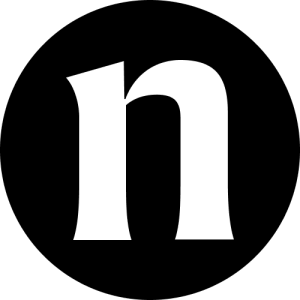
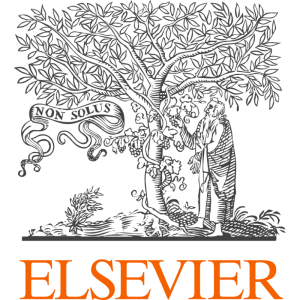
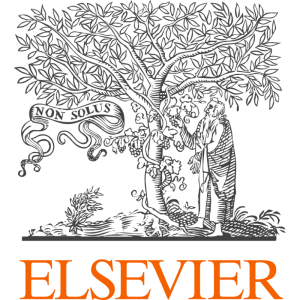